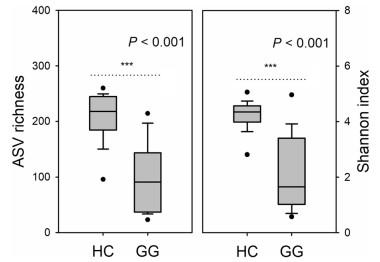
Citation: | Wei Wang, Lizhi Zhou, Rong Fu, Lei Cheng, Shaofei Yan, Nazia Mahtab, Yunwei Song. 2021: Effects of foraging site distances on the intestinal bacterial community compositions of the sympatric wintering Hooded Crane (Grus monacha) and Domestic Duck (Anas platyrhynchos domesticus). Avian Research, 12(1): 20. DOI: 10.1186/s40657-021-00255-8 |
The composition of intestinal microflora in animals is affected by cross-species transmission. In a nature reserve, the foraging sites of waterbirds are relatively fixed, but frequently close to residential areas and can also be visited by domestic fowls. It is easy to result in the trans-species-flock dispersal of gut microbes between the wild birds and domestic fowls. The effects of the variable foraging site distances on the gut microbe structures of the waterbirds and the sympatric domestic fowls are currently unclear, and further research is required to evaluate the impacts of geographic location on cross-infection.
Illumina high-throughput sequencing and bioinformatics analysis software were utilized to compare and analyze the composition of gut microbes from the fecal samples of Hooded Cranes (HC; Grus monacha) and two groups of Domestic Ducks (Anas platyrhynchos domesticus) that foraged at 1 km (ducks in near areas, D-N), and 4 km (ducks in far areas, D-F) away from the habitats of the Hooded Cranes at Shengjin Lake, China.
The results showed that there were significant differences in the alpha-diversity of the gut bacteria in the HC, D-N, and D-F samples under the interspecific distance factor. The dominant bacterial phyla, Cyanobacteria and Proteobacteria, showed correlations with distance for each host. The D-N group had more diverse intestinal flora than the D-F, as they were physically closer to the HC and had more indirect contact and cross-transmission of their gut microbes. More potentially pathogenic bacterial sequences, and Operational Taxonomic Units (OTUs) were found in the D-N than in HC and D-F.
Hooded Cranes and the Domestic Duck populations at variable distances from the cranes showed significant differences in their intestinal bacteria and potentially pathogenic bacteria. The closer the foraging sites were, the easier the intestinal flora spread across species. The results provide a basis for determining the safe distance between wild birds and domestic fowls in a nature reserve.
Empirical studies have demonstrated that gut microbiota play crucial roles for their hosts (Xiang et al. 2019), such as helping digestion (Stanley et al. 2012), improving immunity (Chung et al. 2012) and regulating metabolism (Liu et al. 2020). The intestinal microbiota may even affect hosts' behaviors (e.g. mate choice; Sharon et al. 2010). There might be many factors influenced animal intestinal microbial community patterns, including diet (Bolnick et al. 2014; Fu et al. 2020), life style (Nicholson et al. 2012), genotype (Eckburg et al. 2005; Xiang et al. 2019), gender (Xu et al. 2016; Mason et al. 2019), and seasonal fluctuations (Dong et al. 2019; Zhang et al. 2020).
Migratory birds exhibit unique life history, representing an interesting study object for their gut microbiota. However, there is less information about gut microbiota of wild migratory birds. Hooded Crane (Grus monacha) is a long-distance migratory wild bird, defined as Threatened Species in the International Union for Conservation of Nature and Natural Resources (IUCN) Red List and the first-class national protected wild animal in China. Hooded Crane mainly breeds in south Siberia. From October to next April, they fly to China, Japan and South Korea for wintering (Zheng et al. 2015). Recent studies focused on gut bacterial community structure of Hooded Crane, showing that seasonal dynamics significantly affected gut bacterial diversity (Zhang et al. 2020). Gut bacterial community structure showed dramatic shifts between Hooded Crane and sympatric goose (Xiang et al. 2019; Fu et al. 2020). However, little is known about their gut fungal community. Gut fungi increased nutrition levels for their hosts (Tanahashi et al. 2010, 2017). The roles of gut fungi include provisioning of essential amino acids (Ayayee et al. 2016) and contributing to lignocellulose degradation (Geib et al. 2008; Scully et al. 2012; Herr et al. 2016). Thus, understanding intestinal fungal community might be important for clarifying their ecological function in helping digestion for their hosts.
The Shengjin Lake, an internationally important wetland, is a river-connected shallow lake in the middle of the Yangtze River floodplain. The Shengjin Lake is an important wetland for migratory birds, as it can offer suitable feeding habitats in wintering period. However, the lake is facing serious degradation by anthropic activity in recent years, decreasing food availability for wintering birds (Yang et al. 2015). The Hooded Crane changed their dietary structure and foraged together with poultry in paddy lands (Fu et al. 2020). There are large number of Domestic Goose (Anser anser domesticus; poultry) in this region. The overlap of foraging niche between Hooded Crane and Greylag Goose (Anser anser)might increase the transmission of their gut microbiota and pathogens.
Hooded Cranes fly long distance and live various habitats, thus they might contact a large range of pathogens. Intestinal pathogens could lead to series diseases in wild birds (Singh et al. 2013). Previous studies have demonstrated that different hosts could mutually transmit intestinal pathogens in an overlapping environment through physical contact, air, water, soil, food, or other media (Alm et al. 2018; Fu et al. 2020). Thus, those pathogens in Hooded Cranes could spread to conspecifics and/or sympatric poultry, and vice versa (Altizer et al. 2011). However, the assumption of the cross infection of pathogens between migratory birds and poultry has not been verified. In this study, high-throughput sequencing method (Illumina MiSeq) was used to compare the gut fungal community structure between wintering Hooded Crane and sympatric Greylag Goose, and infer the potential fungal pathogens of each species at the Shengjin Lake.
The Shengjin Lake is a river-connected lake, serving as indispensable wintering and stopover habitat for migratory birds on the East Asia-Australasian flyway (Fox et al. 2011). Hooded Cranes and Greylay Geese often foraged together in paddy fields (Fu et al. 2020). Hooded Crane mainly eats Vallisneria natans and Potamogeton malaianus (Zheng et al. 2015). Artificial hurl food (i.e., paddy) was the main food resource for Domestic Goose. Fecal samples of Hooded Crane and Greylag Goose were collected on December 10, 2018 at the Shegan region, Shengjin Lake. Before sampling, we searched the flocks of Hooded Crane and adjacent Greylag Goose in paddy land. The fresh fecal samples of Hooded Cranes were collected after foraging. The distance among fecal samples was > 5 m to avoid individual repetition. The fecal samples of Domestic Geese (i.e., these geese had overlap foraging niche with Hooded Cranes in paddy field) were collected after artificial hurl food in the yard of farmer's house. The fecal samples were immediately transported to the lab (i.e., within 12 h) and stored at − 20℃.
Fecal DNA was extracted by the Qiagen DNA Stool Mini Kit according to the DNA isolation protocol. The extracted DNA was dissolved in 60μL of elution buffer, quantified by NanoDrop ND-1000 (Thermo Scientific, USA), and stored at − 20℃.
The COL gene was amplified with primer BIRDF1/BIRDR1 (Hebert et al. 2004). The detailed information about PCR reaction was showed in our previous study (Xiang et al. 2019). The amplicon was sequenced and blasted (> 99% identity) in National Center for Biotechnology Information (NCBI) to identify bird species. We only kept these samples belonged to Hooded Crane for the downstream analysis. The fecal samples of Domestic Goose were collected under certain circumstance (i.e., without disturbance of other species), so we need not perform determination for Domestic Goose.
A total of 36 fecal samples (each species with 18 samples) were used in this study. PCR was performed using primer ITS1/ITS2 in 50μL mixtures with parameters as follows: 35 cycles of denaturation at 95℃ for 45 s, annealing at 56℃ for 45 s, and extension at 72℃ for 45 s; with a final extension at 72℃ for 10 min. The PCR products were purified for sequencing.
Fungal raw data were processed by QIIME (v2-2020.2; Bolyen et al. 2019). The deblur algorithm was used to filter the low quality sequences (Amir et al. 2017). Sequences were grouped into amplicon sequence variants (ASVs). The chimeras were filtered by VSEARCH method. Taxonomy was annotated to each ASV using the UNITE database (2020-02-20). Singletons were filtered for downstream analysis. A subset of 40, 000 sequences per sample was chosen to compare fungal community for all samples.
The differences in fungal community compositions between the two hosts were shown by non-metric multidimensional scaling (NMDS) and analysis of similarity (ANOSIM; permutations = 999) using the vegan package (Version 2.0-2) in R software (v.3.4.3). Identification of gut fungal biomarkers in each species was analyzed by linear discriminant analysis (LDA) effect size (LEfSe; Segata et al. 2011). Indicator analysis was conducted to show the enriched genera in each host. The fungal functional guilds (i.e., functional group) were assigned using the FUNGuild pipeline, and only these guilds with high confidence ranking (i.e., highly probable and probable) were selected for further analysis (Nguyen et al. 2016). One-way ANOVA was conducted to evaluate the differences of fungal alpha-diversity (normal distribution, Kolmogorov–Smirnov test) between the two hosts. The Mann–Whitney–Wilcoxon test was performed to compare the relative abundance of animal pathogen and pathogenic diversity (non-normal distribution) between the two hosts.
A total of 2, 116, 221 quality-filtered fungal sequences were obtained across all samples, ranging from 40, 701 to 72, 417 sequences per sample. A total of 1052 fungal ASVs were found, ranging from 23 to 260 across all samples, 25.3% of which (266) shared in guts of the two species (Additional file 1: Fig. S1). The unique gut fungal ASVs were 591 (56.2%) and 195 (18.5%) for Hooded Crane and Greylag Goose, respectively. Gut fungal alpha-diversity (i.e., ASV richness and Shannon index) was significantly higher in Hooded Crane than Greylag Goose (Fig. 1).
The dominant fungal phyla were Ascomycota (72%), Basidiomycota (22%), Zygomycota (3.6%) and Rozellomycota (1.7%) in guts of the two hosts. The Greylag Goose had significantly higher relative abundance of Ascomycota, lower relative abundance of Basidiomycota, Zygomycota and Rozellomycota relative to Hooded Crane (Additional file 1: Fig. S2). Dramatic shifts of gut fungal community structures were detected between Hooded Crane and Greylag Goose (ANOSIM: P = 0.001; Fig. 2). The FUNGuild analysis showed that the guts of Hooded Crane had higher relative abundance of plant saprotroph than Greylag Goose (Additional file 1: Fig. S3).
The LEfSe showed that fungi in four phyla (i.e., Basidiomycota, Glomeromycota, Rozellomycota and Zygomycota), six classes (i.e., Dothideomycetes, Leotiomycetes, Sordariomycetes, etc.), and 14 orders (i.e., Helotiales, Pleosporales, Thelebolales, etc.) were enriched in guts of Hooded Crane (Fig. 3). Fungi from one phylum (i.e., Ascomycota), one class (i.e., Eurotiomycetes) and four orders (i.e., Botryosphaeriales, Dothideales, Eurotiales and Wallemiales) were more abundant in guts of Greylag Goose (Fig. 3). Indicator analysis showed that there were 18 (e.g. Acremonium, Phoma, Rhodotorula, etc.) and six (i.e., Aspergillus, Talaromyces, Sagenomella, etc.) indicator genera in Hooded Crane and Greylag Goose, respectively (Additional file 2: Table S1).
The FUNGuild analysis was used to infer the potentially animal fungal pathogens in Hooded Crane and Greylag Goose. There were 42 potentially pathogenic ASVs across all samples, ranging from zero to ten ASVs per sample. The 17% (i.e., 7) of total potentially pathogenic ASV was detected in both host species (Fig. 4a). The guts of Hooded Crane (i.e., 22) had more unique pathogenic ASV than Greylag Goose (i.e., 13; Fig. 4a). The Mann–Whitney–Wilcoxon test showed that relative abundance of potential pathogen and pathogenic diversity (i.e., ASV richness) were significantly higher in Hooded Crane than Greylag Goose (Fig. 4b, c).
In this study, divergence in the gut fungal community composition and alpha diversity was found between wintering Hooded Crane and Domestic Goose (Figs. 1, 2, 3). Previous studies have shown that diet was the primary driver inducing shifts in microbial community between hosts (Bolnick et al. 2014; Palamidi and Mountzouris 2018). Hooded Crane mainly ate wild Vallisneria natans and Potamogeton malaianus, while artificial hurl food (i.e., paddy) was the main food resource for Domestic Goose (Zheng et al. 2015; Fu et al. 2020). Thus, the dramatic variations in intestinal fungal community might be induced by different diets between the two hosts (Stanley et al. 2012).
The Hooded Crane had higher gut fungal diversity than Greylag Goose (Fig. 1). Recent studies have demonstrated that the external living environment affects animal gut microbial diversity (Rothschild et al. 2018; Perofsky et al. 2019; Schmidt et al. 2019). As Hooded Crane is a kind of migratory bird, which flies long distance and lives at various habitats. However, the living condition and food resources of domestic Greylag Goose were relatively stable. Thus, the more input of environmental microbiota led to higher gut fungal diversity in Hooded Crane than Greylag Goose. A previous study verified that gut microbial diversity was a crucial factor affecting host's digestion and glycemic control (Liu et al. 2020). The wild food resources exhibit lower nutrient contents relative to paddy. Therefore, the higher intestinal fungal diversity might benefit wintering Hooded Cranes to efficiently acquire nutrients from indigestible food (Cantarel et al. 2012; Li et al. 2019).
Furthermore, the higher relative abundance of plant saprotroph was detected in Hooded Crane than Greylag Goose (Additional file 1: Fig. S3). The roots and leaves of certain plants were eaten by Hooded Crane during wintering period (Zheng et al. 2015), thus higher abundance of plant saprotroph might be associated with faster materials conversion and higher nutrient absorption for Hooded Crane. The Acremonium and Rhodotorula were indicator genera in guts of Hooded Crane (Additional file 2: Table S1). Previous studies have demonstrated that the two genera might increase host's digestion and immunity (Li et al. 2018; Chen et al. 2020). The wintering Hooded Crane is under great pressure with food shortage and pathogen invasion (Xiang et al. 2019). Together all, the results suggested that Hooded Crane might depend more on their intestinal microbiota to acquire nutrients and enhance immunity.
In this study, Hooded Crane carried more gut animal pathogenic abundance and diversity than Greylag Goose (Fig. 4), suggesting that wild migratory birds might suffer various pathogens under harsh living conditions. Hooded Cranes are the threatened species, so more work should be done to protect them (Xiang et al. 2019). The 17% of total potentially pathogenic ASVs were detected in both hosts (Fig. 4), suggesting that there might be a little bit of cross infection of fungal pathogens between Hooded Crane and Greylag Goose. As anthropic activities induced the degradation of the lake, the serious reduction in food availability for wintering wild birds happened in recent years (Yang et al. 2015). The wintering birds often foraged together with poultry in paddy fields (Fu et al. 2020). Thus, we hypothesized that the cross-infection of pathogens between Hooded Crane and Greylag Goose might occur when they flocked together for foraging.
There were 42 fungal pathogenic ASVs in Hooded Crane and Greylag Goose (Fig. 4). They could propagate their gut pathogens and increase risk of diseases in other sympatric animals (Ekong et al. 2018; Xiang et al. 2019). Local residents have a lot of contact with domestic poultry, thus those pathogens in guts of Greylag Goose could propagate to human beings. Furthermore, we found two genera with high relative abundance (i.e., Aspergillus and Talaromyces) in guts of Greylag Goose (Additional file 2: Table S1). The Aspergillus could produce ochratoxin (i.e., cancerogen; Cafsi et al. 2020) and the Talaromyces induces enterobrosis (Zhao et al. 2020) for human beings. Thus, the intestinal pathogens in poultry might affect the health of human beings.
The results demonstrated that gut fungal community composition and diversity showed significant difference between wintering Hooded Crane and domestic Greylag Goose. The Hooded Crane might depend more on their gut fungal community to acquire nutrients from indigestible food resources. The Hooded Crane lives at various habitats under harsh living conditions, leading to higher gut fungal pathogenic diversity. Our study also implied that more research should focus on intestinal pathogens in wild birds and domestic poultry, as these pathogens might cause disease in other animals, even human beings. This work helps our further understanding in intestinal microbial community of migratory birds and domestic poultry. However, there were certain limitations. We did not clearly verify the cross infection of gut pathogens between wild birds and domestic poultry along wintering timescale. In addition, the gut fungal communities of Hooded Crane and domestic poultry were studied within one wintering region rather than across multiple regions. The limitations should be clarified in future studies.
The online version contains supplementary material available at https://doi.org/10.1186/s40657-020-00238-1.
We thank Mr. Wei Wang from Anhui University for assistance in sample collection, and Prof. Binghua Sun from Anhui University for assistance with manuscript revision.
XX and ZF designed the experiment; JL, YZ and ZN collected the samples; XX and JL performed the experiments; XX, YZ and ZF performed data analysis; XX and ZF wrote the manuscript; JL, YZ and ZN revised the manuscript. All authors read and approved the final manuscript.
The raw data were submitted to the Sequence Read Archive (SRA) under the accession number SRP276474.
Non-invasive sample collection without hunting of experimental animals was used in this study. Permission was obtained from the Shengjin Lake National Nature Reserve.
Not applicable.
The authors declare that they have no competing interests.
Anderson MJ, Walsh DCI. PERMANOVA, ANOSIM, and the mantel test in the face of heterogeneous dispersions: what null hypothesis are you testing? Ecol Monogr. 2013;83: 557–74.
|
Cairns J, Moerman F, Fronhofer EA, Altermatt F, Hiltunen T. Evolution in interacting species alters predator life-history traits, behaviour and morphology in experimental microbial communities. Proc Biol Sci. 2020;287: 20200652.
|
Elmberg J, Berg C, Lerner H, Waldenström J, Hessel R. Potential disease transmission from wild geese and swans to livestock, poultry and humans: a review of the scientific literature from a One Health perspective. Infect Ecol Epidemiol. 2017;7: 1300450.
|
Holman DB, Bearson BL, Allen HK, Shippy DC, Loving CL, Kerr BJ, et al. Chlortetracycline enhances tonsil colonization and fecal shedding of multidrug-resistant Salmonella enterica serovar Typhimurium DT104 without major alterations to the porcine tonsillar and intestinal microbiota. Appl Environ Microbiol. 2019;85: e02354-e2418.
|
Huang R, Ju Z, Zhou PK. A gut dysbiotic microbiota-based hypothesis of human-to-human transmission of non-communicable diseases. Sci Total Environ. 2020a;745: 141030.
|
Huang SM, Wu ZH, Li TT, Liu C, Han DD, Tao SY, et al. Perturbation of the lipid metabolism and intestinal inflammation in growing pigs with low birth weight is associated with the alterations of gut microbiota. Sci Total Environ. 2020b;719: 137382.
|
Nilsson L, Aronsson M, Persson J, Månsson J. Drifting space use of common cranes—is there a mismatch between daytime behaviour and management? Ecol Indic. 2018;85: 556–62.
|
Revolledo L, Ferreira AJP, Mead GC. Prospects in Salmonella control: competitive exclusion, probiotics, and enhancement of avian intestinal immunity. J Appl Poultry Res. 2006;15: 341–51.
|
Segata N, Izard J, Waldron L, Gevers D, Miropolsky L, Garrett WS, et al. Metagenomic biomarker discovery and explanation. Genome Biol. 2011;12: R60.
|
Wang C, Wang L, Deng DG, Zhou ZZ. Temporal and spatial variations in rotifer correlations with environmental factors in Shengjin Lake. China Environ Sci Pollut Res Int. 2016;23: 8076–84.
|
Wang WJ, Fraser JD, Chen JK. Wintering waterbirds in the middle and lower Yangtze River floodplain: changes in abundance and distribution. Bird Conserv Int. 2017;27: 167–86.
|
Yang ZQ, Zhou LZ. Is intestinal bacterial diversity enhanced by trans-species spread in the mixed-species flock of hooded crane (Grus monacha) and bean goose (Anser fabalis) wintering in the lower and middle Yangtze River floodplain? Animals. 2021;11: 233.
|
1. | Erni Jumilawaty, Yunasfi, Erman Munir, et al. Identification of microplastics in the digestive tract of Great Egret (Egretta alba) in Percut Sei Tuan, North Sumatra. IOP Conference Series: Earth and Environmental Science, 2025, 1445(1): 012117. DOI:10.1088/1755-1315/1445/1/012117 |
2. | Annie G. West, Andrew Digby, Michael W. Taylor. The mycobiota of faeces from the critically endangered kākāpō and associated nest litter. New Zealand Journal of Zoology, 2025, 52(2): 171. DOI:10.1080/03014223.2023.2170428 |
3. | Jing Yin, Dandan Yuan, Ziqiu Xu, et al. Significant Differences in Intestinal Bacterial Communities of Sympatric Bean Goose, Hooded Crane, and Domestic Goose. Animals, 2024, 14(11): 1688. DOI:10.3390/ani14111688 |
4. | Zeng Jiang, Mingqin Shao, Jianying Wang. Simulation of Spatial and Temporal Patterns of Suitable Wintering Habitat for Hooded Crane (Grus monacha) Under Climate and Land Use Change Scenarios. Animals, 2024, 15(1): 6. DOI:10.3390/ani15010006 |
5. | Nazia Mahtab, Yuannuo Wu, Jing Yin, et al. Comparison of the gut fungal communities among Hooded crane (Grus monacha), Greater white-fronted goose (Anser albifrons), and Bean goose (Anser fabalis) at Shengjin Lake, China. Global Ecology and Conservation, 2024, 49: e02767. DOI:10.1016/j.gecco.2023.e02767 |
6. | Patthanan Sakda, Xingjia Xiang, Yuannuo Wu, et al. Gut Fungal Communities Are Influenced by Seasonality in Captive Baikal Teal (Sibirionetta formosa) and Common Teal (Anas crecca). Animals, 2023, 13(18): 2948. DOI:10.3390/ani13182948 |
7. | Yuannuo Wu, Xiaoyu Fan, Jie Yu, et al. Characteristics of cross transmission of gut fungal pathogens between wintering Hooded Cranes and sympatric Domestic Geese. Avian Research, 2023, 14: 100142. DOI:10.1016/j.avrs.2023.100142 |
8. | Yunzhu Liu, Lan Wu, Jia Guo, et al. Habitat selection and food choice of White-naped Cranes (Grus vipio) at stopover sites based on satellite tracking and stable isotope analysis. Avian Research, 2022, 13: 100060. DOI:10.1016/j.avrs.2022.100060 |
9. | Yuannuo Wu, Zihan Li, Jingru Zhao, et al. Significant differences in intestinal fungal community of hooded cranes along the wintering periods. Frontiers in Microbiology, 2022, 13 DOI:10.3389/fmicb.2022.991998 |
10. | Jingjing Gu, Lizhi Zhou. Intestinal Microbes of Hooded Cranes (Grus monacha) Wintering in Three Lakes of the Middle and Lower Yangtze River Floodplain. Animals, 2021, 11(5): 1390. DOI:10.3390/ani11051390 |
11. | Nazhong Zhang, Lizhi Zhou, Zhuqing Yang, et al. Effects of Food Changes on Intestinal Bacterial Diversity of Wintering Hooded Cranes (Grus monacha). Animals, 2021, 11(2): 433. DOI:10.3390/ani11020433 |
12. | Nazia Mahtab, Lizhi Zhou, Fengling Zhang, et al. Seasonal Variations in the Gut Fungal Communities of Hooded Crane (Grus monacha) at Wintering and Stopover Sites in China. Animals, 2021, 11(4): 941. DOI:10.3390/ani11040941 |