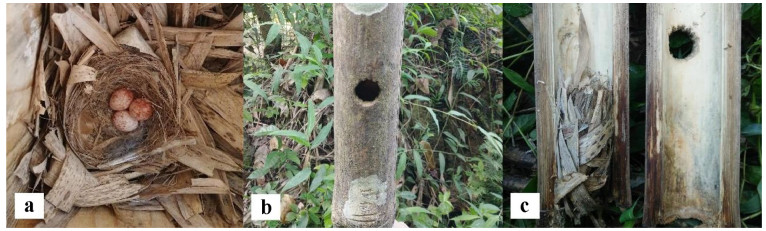
Citation: | Kevin A. Wood, Phoebe Ham, Jake Scales, Eleanor Wyeth, Paul E. Rose. 2020: Aggressive behavioural interactions between swans (Cygnus spp.) and other waterbirds during winter: a webcam-based study. Avian Research, 11(1): 30. DOI: 10.1186/s40657-020-00216-7 |
Our understanding of any impacts of swans on other waterbirds (including other swans), and potential effects on waterbird community structure, remain limited by a paucity of fundamental behavioural and ecological data, including which species swans interact aggressively with and how frequently such interactions occur.
Behavioural observations of aggression by swans and other waterbirds in winters 2018/2019 and 2019/2020, were carried out via live-streaming webcams at two wintering sites in the UK. All occurrence sampling was used to identify all aggressive interactions between conspecific or heterospecifics individuals, whilst focal observations were used to record the total time spent by swans on aggressive interactions with other swans. Binomial tests were then used to assess whether the proportion of intraspecific aggressive interactions of each species differed from 0.5 (which would indicate equal numbers of intraspecific and interspecific interactions). Zero-inflated generalized linear mixed effects models (ZIGLMMs) were used to assess between-individual variation in the total time spent by swans on aggressive interactions with other swans.
All three swan species were most frequently aggressive towards, and received most aggression from, their conspecifics. Our 10-min focal observations showed that Whooper (Cygnus cygnus) and Bewick's Swans (C. columbianus bewickii) spent 13.8± 4.7 s (means± 95% CI) and 1.4± 0.3 s, respectively, on aggression with other swans. These durations were equivalent to 2.3% and 0.2% of the Whooper and Bewick's Swan time-activity budgets, respectively. Model selection indicated that the time spent in aggressive interactions with other swans was best-explained by the number of other swans present for Whooper Swans, and an interactive effect of time of day and winter of observation for Bewick's Swans. However, the relationship between swan numbers and Whooper Swan aggression times was not strong (R2= 19.3%).
Whilst swans do exhibit some aggression towards smaller waterbirds, the majority of aggression by swans is directed towards other swans. Aggression focused on conspecifics likely reflects greater overlap in resource use, and hence higher potential for competition, between individuals of the same species. Our study provides an example of how questions relating to avian behaviour can be addressed using methods of remote data collection such as live-streaming webcams.
Avian reproduction has a series of complex behaviors, such as mate selection, nest site selection, egg laying, incubation, and nestling feeding (Zheng 2012). These behaviors are a sequence of adaptations formed through natural selection that are beneficial to individual and population survival (Saether and Bakke 2000). There are about 10, 000 bird species (del Hoyo et al. 2013). The fundamental breeding information (nesting, incubation and nestling periods) of only about one-third of these species is known while the rest are poorly understood (Xiao et al. 2017). Passerines have the greatest number of species (6004) and inhabit all continents except Antarctica (Sibley et al.1991; del Hoyo et al. 2013). Fewer than 7% of studies on passerines include breeding data (Pienaar et al. 2013). The tropics contain 73% of all bird species but breeding information have been well documented in only 20.2% of tropic bird species, compared to 57.9% of bird species in temperate areas (Kricher 2001; Levy 2008; Xiao et al. 2017). It is difficult to study the natural habitats and nests of birds in tropical forests because of the hot and humid climate and dense vegetation (Xiao et al. 2017). Using artificial nest boxes facilitates studies of breeding biology of birds, especially for secondary cavity-nesting birds. Detailed information of avian reproduction can be obtained when birds accept and use nest boxes. Artificial nest boxes are convenient for breeding ecology studies but are also useful for other studies such as niche utilization (Ye et al. 2019).
We studied the Yellow-bellied Warbler (Abroscopus superciliaris) (Passeriformes, Cettiidae). There are three species in Abroscopus, including the Yellow-bellied Warbler, Rufous-faced Warbler (A. albogularis) and Black-faced Warbler (A. schisticeps). Knowledge of the breeding ecology of these three warblers is limited and little clutch size information has been reported (Xiao et al. 2017). We studied the breeding ecology of A. superciliaris and documented reproductive information throughout egg and nestling periods. Data collected included nest components, nest size, egg laying date and time, egg morph, egg size, clutch size, egg incubation, nestling brooding and feeding, nestling morph and growth, and reproductive outcome.
This study was performed in Nonggang village (23°39ʹN, 107°04ʹE), located in Guangxi Province of Southwest China, from April to July 2019. This location has a tropical monsoon climate with an average annual temperature ranging from 20.8–22.4℃ and an average annual rainfall ranging from 1150–1550 mm (Feng et al.2019). A. superciliaris are resident birds mainly distributed in the eastern part of the Himalayas, Southern China and Southeast Asia. They are secondary cavity-nesters with similar morphology between males and females (del Hoyo et al. 2013).
We established 191 nest boxes to attract A. superciliaris. Nest boxes were made from pine wood and were 30 cm high × 15 cm long and wide, with a 6-cm diameter hole. They were attached to trees at a height of 3 m before the breeding season and inspected regularly for warbler activity. Nests in natural habitat were found by searching for holes in bamboo habitat. We recorded the nest materials, egg laying date, egg morph, clutch size, nestling morph, and measured the egg size, egg mass, nestling tarsus length, and nestling mass during nest inspection. Egg length, egg width, and nestling tarsus length were surveyed by a caliper. Egg mass and nestling mass were surveyed by an electric scale. For the calculation of egg size and mass, to avoid pseudo-replication, the average values from each nest represented one sample rather than using each egg as one sample. Two warbler nests in nest boxes were monitored 24 h continuously from egg laying to egg incubation and nestling feeding stages using a mini-camera (WJO3, Hisilicon, Shenzhen, P.R. China). A total of 77, 760 min of video were recorded from nest T12 and J1. T12 included intact video data of both egg and nestling stages for analyses while for J1 only video data of nestling stage was included because the video data of egg stage was incomplete and excluded from analyses.
The egg laying date and time, egg incubation and nestling feeding behaviors were extracted by studying the monitoring videos. We summarized incubation rate and time per hour from 6:00 to 19:00 and daily incubation rate and time during egg incubation stage. During the nestling stage, we summarized daily brooding (i.e. a behavior that keeps nestlings warm) frequency and time, feeding frequency per hour from 6:00 to 19:00, and daily feeding frequency. The evaluation of reproductive outcome included calculations of hatching rate, fledging rate, fledging success, and nesting success. Hatching rate was calculated by the proportion of hatching eggs from all eggs while fledging rate was calculated by the proportion of fledglings from all nestlings. Nesting success was defined as the percentage of nests that fledged at least one young and fledging success was calculated as the brood sizes at fledging (Yang et al. 2011). Figures were generated using SigmaPlot 14.0 for Windows (Systat Software Inc., USA). A Student's t test was used to compare the nest sizes in the nest boxes and natural nests. Spearman's rank correlation coefficient was used to test the correlation between incubation rate and time or egg turning frequency. Logistic regression was used to fit the growing curve of nestlings. Data were presented as mean ± SD. Data analyses were performed using IBM SPSS 25.0 for Windows (IBM Inc., USA).
We found a total of 10 nests of A. superciliaris in nest boxes and 6 nests in natural habitats. Detailed information on breeding ecology was acquired from the nest boxes. We were unable to collect further information except for habitat, nest size, and nest fate because these nests were disturbed by local inhabitants. The nests of A. superciliaris in nest boxes were composed of an awn weaving nest cup surrounded by dry bamboo leaves. The nest cup included some feathers (Fig.1a). In natural habitat A. superciliaris built nests in bamboo holes (hole diameter: 2.5 ± 0.2 cm, n = 6) that were created by the White-browed Piculet (Sasia ochracea) (Fig.1b). The holes were 1.27 ± 0.27 m (n = 6) high. The nest materials were similar to those in the nest boxes but the nest shape differed (Fig.1c). The nest cup diameter was similar between nest box and bamboo nests (nest box: 5.3 ± 1.1 cm, n = 6, bamboo: 5.1 ± 1.5 cm, n = 6; t = -0.359, df = 10, P = 0.727, Student's t-test), but the nest cup depth was different (nest box: 4.2 ± 0.3 cm, n = 6, bamboo: 3.4 ± 0.4 cm, n = 6; t = -3.839, df = 10, P = 0.003, Student's t-test). Clutch size was 4.3 ± 1.2 (range: 2–6, n = 10) and eggs were white in ground color but densely covered with brownish-red markings, which were mostly present on the blunt end (Fig.1a). Egg size and mass were 14.66 ± 0.54 mm × 11.03 ± 0.30 mm and 0.93 ± 0.11 g (n = 7), respectively.
Nest box data showed that the earliest and latest laying dates of the 1st egg were 24 April and 31 May, respectively. A. superciliaris laid one egg each day during egg laying. The laying time of three eggs (3rd, 4th, and 5th egg) from nest T12 was recorded on 6:52, 6:18, and 6:54 in the morning. The egg incubation began after the last egg was laid. Before that, a short-time incubation was also found during the egg laying stage (Fig.2). Although male and female warblers were sexually monomorphic, incubation appeared to be performed solely by the female. The incubation duration and absence intervals showed no signs of alternate incubation. The daily incubation rate was high and the duration was long initially but decreased during the latter part of the egg stage. On the day before the hatch of the first nestling, the incubation time increased to a peak (Fig.2). Incubation rate was positively correlated with incubation time and egg turning frequency (frequency vs time: r = 0.664, P = 0.007; frequency vs turning: r = 0.606, P = 0.017, Spearman's rank correlation coefficient). The incubation time per hour of a day from 6:00 to 19:00 was relatively longer in the morning compared to afternoon, but increased on the last hour (18:00–19:00) (Fig.3). Compared with incubation time, incubation rate was more variable during the incubation period (Fig.3). The egg incubation stage lasted 13 ± 1.7 days (range: 12–15, n = 3), and females remained in the nest boxes at night during the entire incubation stage (701.5 ± 26.3 min/night, n = 12).
The hatching time of two nestlings in nest T12 was 6:55 and 6:26 on the mornings of two successive days. For nest J1, the hatching time of two nestlings was 11:35 and 12:55 on the same day. Eggshells were ejected by females after nestlings hatched. Females sat on the nest after nestlings hatched to keep the nestling warm (i.e., nestling brooding). The brooding time lasted 11 days, which accounted for 68.75% of nestling stage. It was high on the first 3 days, but decreased rapidly by the 6th day and was absent on the last 5 days (Fig.2). The female parent remained in the nest box at night (649.9 ± 37.8 min/night, n = 10) until the end of nestling brooding (Fig.4). Both males and females participated in nestling feeding. The feeding frequencies of T12 and J1 were similar. They increased gradually with nestling growth but decreased on the last 2 days (Fig.4). The feeding frequency per hour of a day from 6:00 to 19:00 had minor fluctuations (Fig.3).
The growth curves of tarsus length and body mass in nestlings were fitted by logistic regression (Fig.5). Hatchlings on 0 d were naked with some fluffy down on the head. Their skin was a reddish color on 0 d but was yellowish from 1 d forward (Fig.6). Feather buds appeared on the forelegs and the center of the back at 4 d and began to grow within capsules. From 13 d the wing feathers had emerged from the capsules and at the time of fledging the nestling was similar in color to that of adult bird with a white brow and yellow belly (Fig.6). The nestling stage lasted 13.7 ± 1.5 days and ranged from 12 to 15 days (n = 3).
In the 10 nest box nests, the hatching and fledging rates of A. superciliaris were 25.58% (11 out of 43) and 54.55% (6 out of 11), respectively. Occupation by White-rumped Shama (Kittacincla malabarica), predation, and desertion led to nest failure. The nesting success was 40%, with an average fledging success of 1.1 fledglings per nest. All nests from bamboo holes successfully fledged, with a nesting success of 100%, but other information including eggs and nestlings were not studied.
The incubation time of A. superciliaris gradually decreased during the latter part of the egg stage, but significantly increased on the day before the first nestling hatched (Fig.2). Female parents spent much more time in the nest immediately before the egg hatch. The parent could perceive that the eggs were near hatch. Egg embryos are sensitive to ambient temperature but tolerance to temperature can vary during different development stages (Webb 1987). The parent may modify its incubating behavior according to the development of the egg embryo. Several studies have documented parent-embryo communication in birds (Colombelli-Négrel et al. 2012; Mariette and Buhanan 2016; Katsis et al. 2018; Mariette et al. 2018). It is possible that A. superciliaris parents can either perceive the progress of embryo development or communicate with the embryo to achieve synchronism. Further studies are needed to test these hypotheses.
According to the allocation of incubation time per hour during the day, females invested more time in the morning than in the afternoon (Fig.3). This suggests that parental foraging time mainly occurred in the afternoon. Birds balance their own survival and reproduction during breeding season (Dammhahn et al. 2018); therefore, such pattern of time allocation may be the result of tradeoff between parent survival and embryo development. Allocating more time for foraging in the afternoon is reasonable because the temperature may be warmer for egg embryos than in the morning. Although the incubation time was similar between some time intervals, the incubation rate was not necessarily consistent. For example, the incubation time between 6:00–7:00 and 18:00–19:00 was similar, but the incubation rate of the former was lower than the latter (Fig.3). This suggests that the parent can maintain the incubation time by modifying the incubation rate. The parent may allocate more time per incubation during 6:00–7:00 than during 18:00–19:00, but maintain a similar total incubation time. This is reasonable because during the last hour before nightfall, parents may come out more frequently to search for food.
This study provides information on the breeding ecology of A. superciliaris. The breeding ecology of the other two species in Abroscopus is poorly known. According to the phylogeny of old-world warblers, the most closely related warbler clades are Cettia, Tesia, and Urosphena (Alström et al. 2011; Jetz et al. 2012). However, the breeding ecology of only two Cettia warblers (C. diphone and C. cetti) is known (Xiao et al. 2017). Unlike A. superciliaris, these warblers generally built nests in shrubby vegetation rather than in cavities (del Hoyo et al. 2013). For Cettia warblers, egg incubation and nestling feeding are solely performed by females (Kenzo and Takeshi 1970; Bibby 1982; Tasinazzo 1993; del Hoyo et al. 2013), In A. superciliaris males did not incubate eggs but they did participate in nestling feeding. Further studies on the breeding ecology of the other two Abroscopus species might help us to understand their evolution in relation to A. superciliaris.
We thank anonymous reviewers for their helpful comments on an earlier version of this manuscript. We thank Accdon for linguistic assistance during manuscript preparation.
CY conceived and designed the study. JB and YJ conducted the experiments in the field. JB and CY performed the data analyses and wrote the manuscript. All authors read and approved the final manuscript.
This article does not contain any studies with human participants performed by any of the authors. The experiments comply with the current laws of China.
Not applicable.
The authors declare that they have no competing interests.
Beekman J, Koffijberg K, Wahl J, Kowallik C, Hall C, Devos K, et al. Long-term population trends and shifts in distribution for Bewick's Swans Cygnus columbianus bewickii wintering in northwest Europe. Wildfowl. 2019; Special Issue 5: 73–102.
|
Beven G. Coot feeding on weed disturbed by Mute Swans. Brit Birds. 1980;73:219–20.
|
Bowler JM. Feeding strategies of Bewick's Swans (Cygnus columbianus bewickii) in winter. PhD Thesis. Bristol: University of Bristol; 1996.
|
Crawley MJ. The R Book. 2nd ed Chichester: Wiley; 2013.
|
Frost TM, Calbrade NA, Birtles GA, Mellan HJ, Hall C, Robinson AE, et al. Waterbirds in the UK 2018/2019: The Wetland Bird Survey. Thetford: BTO/RSPB/ JNCC; 2020.
|
Gurtovaya EN. Aggressive interactions between Bewick's Swans and other Anseriformes in the breeding period. Casarca. 2000;6:167–76.
|
Johnsgard PA. Handbook of waterfowl behavior. New York: Cornell University Press; 1965.
|
Lumsden HG. Trumpeter Swans and Mute Swans compete for space in Ontario. Ontario Birds. 2016;34:14–23.
|
Newth JL, McDonald RA, Wood KA, Rees EC, Semenov I, Chistyakov A, et al. Predicting intention to hunt protected wildlife: a case study of Bewick's swans in the European Russian Arctic. Oryx. (in press).
|
R Core Team. R: A language and environment for statistical computing. [3.6.3]. Vienna, Austria: R Foundation for Statistical Computing; 2020.
|
Rees EC. Bewick's Swan. London: T & AD Poyser; 2006.
|
Rees EC, Cao L, Clausen P, Coleman JT, Cornely J, Einarsson O, et al. Conservation status of the world's swan populations, Cygnus sp. and Coscoroba sp.: a review of current trends and gaps in knowledge. Wildfowl. 2019; Special Issue 5: 35–72.
|
Scott DK. Social behaviour of wintering Cygnus columbianus bewickii. In: Matthews GVT, Smart M, editors. Proceedings of the Second International IWRB Swan Symposium, Sapporo, Japan, 1980. Slimbridge: International Waterfowl Research Bureau; 1981. p. 211–25.
|
Sladen WJL. Swans should not be hunted. In: Sears J, Bacon PJ, editors. Proceedings of the Third International IWRB Swan Symposium, Oxford, 1989. Slimbridge: International Waterfowl Research Bureau; 1991. p. 368–75.
|
Stone WB, Marsters AD. Aggression among captive Mute Swans. New York Fish Game J. 1970;17:51–3.
|
Therres GD, Brinkler DF. Mute Swan interactions with other birds in Chesapeake Bay. In: Perry MC, editor. Mute Swans and Their Chesapeake Bay Habitats: Proceedings of a Symposium. Virginia: US Geological Survey; 2004. p. 43–6.
|
Wood KA, Cao L, Clausen P, Ely CR, Luigujõe L, Rees EC, et al. Current trends and future directions in swan research: insights from the 6th International Swan Symposium. Wildfowl. 2019a; Special Issue 5:1–34.
|
1. | Ziqi ZHANG, Qihong LI, Yan CAI, et al. Revealing the key signals in nestling begging behavior perceived by parent birds during parent–offspring conflict. Integrative Zoology, 2024. DOI:10.1111/1749-4877.12901 |
2. | Ziqi Zhang, Jianli Bi, Xu Zhao, et al. Comparison of Reproductive Strategies between Two Sympatric Copsychus Passerines. Animals, 2024, 14(4): 554. DOI:10.3390/ani14040554 |
3. | Karl T. Heide, Thomas E. Martin. Breeding biology of the Bornean Stubtail (Urosphena whiteheadi) on Mount Kinabalu, Sabah, Malaysia. The Wilson Journal of Ornithology, 2023, 135(2) DOI:10.1676/22-00078 |
4. | Qihong Li, Jianli Bi, Jiangwen Wu, et al. Impact of nest sanitation behavior on hosts’ egg rejection: an empirical study and meta-analyses. Current Zoology, 2021, 67(6): 683. DOI:10.1093/cz/zoab057 |