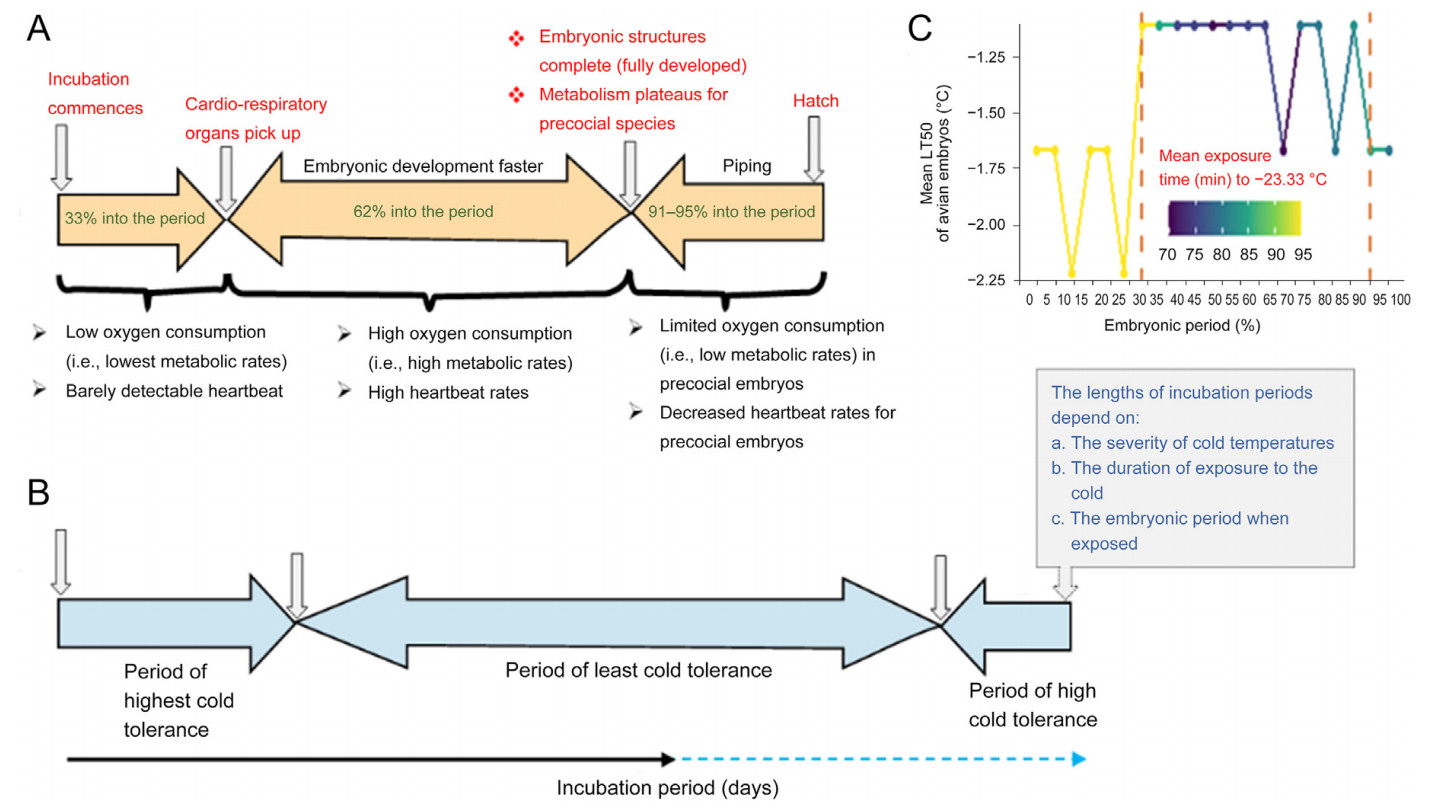
Citation: | Ibrahim M. Ahmad, Dongming Li. 2023: More than a simple egg: Underlying mechanisms of cold tolerance in avian embryos. Avian Research, 14(1): 100104. DOI: 10.1016/j.avrs.2023.100104 |
Avian embryos, which develop within eggs, exhibit remarkable tolerance to extremely low temperatures. Despite being a common trait among all birds, the mechanisms underlying this cold tolerance in avian embryos remain largely unknown. To gain a better understanding of this phenomenon and the coping mechanisms involved, we reviewed the literature on severe cold tolerance in embryos of both wild and domestic birds. We found that embryos of different bird orders exhibit tolerance to severe cold during their development. In response to cold stress, embryos slow down their heartbeat rates and metabolism. In severe cold temperatures, embryos can suspend these processes, entering a torpid-like state of cardiac arrest. To compensate for these developmental delays, embryos extend their regular incubation periods. Depending on their embryonic age, embryos of all bird species can tolerate acute severe cold regimes; only a few tolerate chronic severe cold regimes. We also discussed various extrinsic and intrinsic factors that affect the tolerance of bird embryos to low temperatures before and after incubation. Cold tolerance appears to be a heritable trait shared by wild and domestic embryos of all bird classes, regardless of egg size or development (altricial/precocial). Driven by environmental variability, cold tolerance in avian embryos is an optimal physiological and ecological strategy to mitigate the adverse effects of cold conditions on their development in response to fluctuating environmental temperatures.
Avian embryos develop externally within eggs during incubation, resembling adult ectotherms for the majority of the duration due to limited thermoregulatory capacity (Martin et al., 2007; Andrewartha et al., 2011). As the metabolism of ectotherms, including avian embryos, is heavily reliant on environmental conditions, primarily temperatures, any deviation from optimal ambient or incubation temperatures can lead to hypothermia (in the case of low temperatures) or hyperthermia (in the case of high temperatures) (Nichelmann and Tzschentke, 2002). While avian embryos are quite tolerant of hypothermia, they are highly susceptible to hyperthermia (Batt and Cornwell, 1972; Bennett and Dawson, 1979; Webb, 1987; Divoky and Harter, 2007). Naturally, avian embryos experience deviations from optimal incubation temperatures when incubation is interrupted. This is more so in wild birds, as it is common for eggs to be left unattended for several days before the clutch is completed (Martin et al., 2007; Nord and Nilsson, 2011; Griffith et al., 2016). Also, once incubation begins, the incubating parents leave the nest for periods of time either to forage or avoid predators. These periods of neglect, also known as off-bouts, can result in intermittent cooling of the developing embryos depending on various factors such as the local climate, nest architecture, and duration of the off-bouts. Even in warm environments, unattended eggs may experience some degree of cooling (DuRant et al., 2011). Typically, off-bouts last only a few minutes in warmer environments, while they can last for hours or even days in colder climates (Macdonald et al., 2013).
The cold tolerance capacity of avian embryos is determined by how long they were exposed to cold conditions and whether they hatched after being re-warmed (Ewert, 1991). Both extrinsic and intrinsic factors contribute to the cold tolerance capability of avian embryos. Parent birds have evolved a range of reproductive traits, such as nest-building and incubation behaviors, to provide stable and buffered conditions for embryonic development (Martin et al., 2007; Duan et al., 2019). Still, some avian embryos must endure fluctuating incubation temperatures. These selective pressures (fluctuating temperatures outside the mother’s uterus) have driven them to evolve the ability to slow down or even suspend embryonic development until they detect favorable conditions (Tazawa and Rahn, 1986; Tazawa et al., 1994). They can instantly slow down their metabolism and heartbeat rate (HR) in a species-specific manner when temperatures drop, and if the temperature continues to drop to a species-specific threshold, they eventually enter cardiac arrest (Tazawa and Rahn, 1986; Ar and Tazawa, 1999; Andrewartha et al., 2011). Generally, their capability to tolerate cold conditions reduces with embryonic age; it may slightly increase in precocial species around internal piping stage probably due to endothermy—the ability to regulate body temperature through metabolism-dependent heat production—that develops around hatching. This is not the case in altricial species probably because their endothermy manifests later-on after hatching (Nichelmann and Tzschentke, 2002; Walter and Seebacher, 2009).
The concept of cold tolerance has been a subject of interest for a considerable period of time (Colasanti, 1875). Similar to true ectotherms, avian embryos are unable to regulate their body temperature and exhibit torpor-like conditions when they detect suboptimal low temperatures (Feast et al., 1998; Mundim et al., 2020). They are able to compensate for these developmental delays caused by exposure to cold temperatures by extending their incubation periods to ensure complete embryonic development before hatching. Previous studies have linked ecology and phylogeny to explain the tolerance of bird embryos to severe cold. However, the mechanisms that underlie cold tolerance in avian embryos remain unclear. To improve our understanding of the cold tolerance and the coping mechanisms of avian embryos, this review delves into the ubiquitous phenomenon of cold tolerance in avian embryos. We discussed how avian embryos modulate their heart rate and metabolism in response to cold, and the extrinsic and intrinsic factors that affect their cold tolerance before and during incubation.
Although severe cold stress might affect embryonic viability, avian embryos show resilience and can still hatch (Barrionuevo and Frere, 2012). This is despite the cold stress stretching for several hours or days—typical of cold breeding seasons or temperate and Arctic environments, thereby exposing them to low temperatures below their physiological zero temperatures (24–28 ℃), which are the threshold temperatures for development (Morton and Pereyra, 1985; Stoleson, 1999). For instance, the eggs of the altricial Great Tit (Parus major) can tolerate sudden cold snaps of 2–3 ℃, and a significant proportion of these eggs have been observed to hatch and eventually fledge following this cold treatment (Glądalski et al., 2020). Similarly, the eggs of the altricial European Blackbird (Turdus merula) have successfully hatched after being exposed to a mean temperature of 9 ℃ for 8 h and then rewarmed (Magrath, 1988). Even the precocial Domestic Chicken (Gallus domesticus) embryos have been shown to survive after their internal egg temperature dropped to −1.67 ℃, following an experimental 95-min exposure to a sub-freezing temperature of −23.33 ℃ along their 0–20 day incubation period (Moreng and Shaffner, 1951). Additionally, the eggs of another precocial species, the Mallard (Anas platyrhynchos) have been observed to hatch successfully after exposure to an intermittent 0 ℃ environment (Batt and Cornwell, 1972).
Despite this remarkable cold tolerance, it is crucial to limit their exposure to such conditions. Intermittent periods of optimal incubation temperatures, determined by their evolutionary history, are necessary for their survival. A constant suboptimal temperature can prevent the embryos from hatching successfully. For instance, chicken eggs can hatch successfully if they are incubated at optimal temperatures for the first 80% of their incubation period, followed by a temperature reduction to 33.8 ℃ for the remaining period (Ackman and Seagrave, 1984). Conversely, they will fail to hatch if incubated continuously at 34 ℃ (Mortola, 2006). Similarly, Arctic Fork-tailed Storm-petrel (Oceanodroma furcata) eggs can hatch successfully if incubated at 34 ℃, even with intermittent cooling to 10 ℃, but continuous incubation at 26 ℃ leads to death, though most embryos can reach external piping (Vleck and Kenagy, 1980). The ability of bird embryos to tolerate cold stress is a notable evolutionary adaptation of eggs exposed to land environments, compared to those in relatively stable aquatic systems (Martin and Wiebe, 2004).
Phylogenetically, the embryos of seabirds belonging to the Procellariiformes order, followed by Charadriiformes, have evolved to be the most tolerant to chilling temperatures and prolonged egg neglect (Boersma and Wheelwright, 1979; Boersma et al., 1980; Astheimer, 1991). Apart from the neglect, some of these eggs are laid on ground nests, forcing the embryos to develop on frozen grounds. Despite the neglect and harsh nesting conditions, these embryos eventually hatch. For instance, the eggs of Arctic breeding Fork-tailed Storm-petrels hatched after being neglected for 28 days under a mean burrow temperature of 10 ℃ (Boersma et al., 1980). Similarly, Arctic breeding Black Guillemot (Cepphus grille) eggs hatched when incubation resumed following 14 days of neglect under a daily mean temperature of 2.9 ℃ and nest cavity temperature of <5 ℃. Other species also display remarkable resilience to cold exposures. In the Magellanic Penguin (Spheniscus magellanicus) belonging to order Sphenisciformes, their eggs hatched despite the daily nest temperatures dropping to 11.7 ℃ for 9 h (Barrionuevo and Frere, 2012). It appears that repeated cold exposure does not adversely affect the survival of several avian embryos (Morton and Pereyra, 1985). However, the mechanisms by which the eggs of seabirds and similar avian species survive the long and harsh cold conditions during their incubation periods remain incompletely understood.
The ability of avian embryos to tolerate cold temperatures is determined by three key factors: the severity of the cold, the age of the embryo at the time of exposure, and the duration of exposure (acute or chronic). While these factors work together to determine cold tolerance, the age of exposure and the duration of exposure are the most critical factors (Moreng and Shaffner, 1951; Tazawa and Rahn, 1986; Suarez et al., 1996).
During the early stages of embryonic development, most avian embryos exhibit a greater tolerance to cold temperatures than during later stages, regardless of their phylogeny or ecology (Fig. 1; Andrewartha et al., 2011). This has been observed in various species. The survival rate of Cassin’s Auklet (Ptychoramphus aleuticus) embryos, which were left unattended under 12 ℃, was found to be higher in the first half of their incubation period as opposed to the latter half (Astheimer, 1991). Mallard eggs can hatch when exposed to a one-time 0 ℃ for 10 h from embryonic day (E) 0 to E9 (≤33% of their incubation period); however, these eggs fail to hatch when exposed to the same treatment beyond the initial incubation period (Batt and Cornwell, 1972). White-rumped Munia (Lonchura striata) embryos survived the first 10 days of daily 10 ℃ for 6 h (Zhao et al., 2017). Furthermore, wild birds in cold regions are more likely to neglect their eggs at the beginning of the incubation period, but this frequency decreases as the incubation period progresses (Astheimer, 1991). If avian embryos are exposed to severe cold at early stage, high hatching success can occur even if the intermittent cold exposure persists throughout the incubation period (Barrionuevo and Frere, 2012; Zhao et al., 2017). This suggests that early embryonic age plays a crucial role in determining the ability of avian embryos to tolerate cold exposure.
In order to assess the acute cold tolerance of Domestic Chicken embryos, Moreng and Shaffner (1951) subjected eggs to a single 95-min sub-freezing temperature (−23.33 ℃) along the 0–20 day incubation period (Fig. 1C). They found that early-age embryos took longer to freeze than more advanced embryos: embryos between embryonic day (E) 0–6 (≤33% of their incubation period) were the most tolerant to cold exposure. This indicates that Domestic Chicken (Galliformes) embryos can tolerate acute doses of cold to the same extent as Arctic seabirds, highlighting the importance of cold tolerance as a ubiquitous phenomenon in Aves. What is interesting is that chicken embryos held-on to this trait despite being artificially selected, incubated, and domesticated under optimal conditions for centuries (Hata et al., 2021). The reasons why Domestic Chickens retained the cold tolerance trait are unclear and require further investigation. Although Moreng and Shaffner’s results were astounding, they could have been more ecologically relevant if they had incubated the eggs that managed to survive until hatching. This is because hatching success should be the true determinant of cold tolerance. Therefore, future studies on cold tolerance should extend beyond immediate survival rates to hatching rates.
Many bird species, including chicken and other Phasianidae embryos, show high hatching success after prolonged cold exposure throughout their incubation periods (Table 1). For instance, studies have shown that nearly 100% of Chicken and Japanese Quail (Cortunix japonica) eggs can hatch after being exposed to daily 10 ℃ for 6 h (Zhao et al., 2017). Similarly, 90% of Blood Pheasant (Ithaginis cruentus) eggs can hatch successfully after being neglected daily under 4.5 ℃ for 6.6 h. Likewise, it has been observed that 88% of Sichuan Partridges (Arborophila rufipectus) eggs can hatch successfully after being left unattended daily under 13 ℃ for 4.5 h (Ong-in et al., 2016). Under optimal incubation temperatures, Domestic Chickens and their wild siblings have almost similar incubation periods (Ong-in et al., 2016). Following cold exposure, however, chickens can only extend their incubation periods to 28 days (Fu et al., 2017; Zhao et al., 2017); wild species such as partridges can extend theirs to over 30 days (Ong-in et al., 2016).
Order | Family | Common name | Scientific name | Incubation behavior | Breeding locality | Ambient/experimental temperatures (℃) | Days of egg neglect | Embryonic age of exposure | Duration of cold exposure | Increase in incubation period | Hatching success (%) | References |
Procellariiformes | Hydrobatidae | Fork-tailed Storm-petrel | Oceanodroma furcata | Biparental incubators | East Amatuli Island, Barren Islands, Alaska, USA | 10 | 7.6 days | Spanned the incubation period | Continuous | 9.3 days | 100 | Boersma and Wheelwright (1979) |
Procellariiformes | Hydrobatidae | Fork-tailed Storm-petrel | Oceanodroma furcata | Biparental incubators | East Amatuli Island, Barren Islands, Alaska, USA | 10 | 15.5 days | Spanned the incubation period | Continuous | 17.6 days | 100 | Boersma and Wheelwright (1979) |
Procellariiformes | Hydrobatidae | Fork-tailed Storm-petrel | Oceanodroma furcata | Biparental incubators | East Amatuli Island, Barren Islands, Alaska, USA | 10 | 28 days | Spanned the incubation period | Continuous | 23 days | 100 | Boersma et al. (1980) |
Procellariiformes | Hydrobatidae | Fork-tailed Storm-petrel | Oceanodroma furcata | Biparental incubators | Laboratory | 26 | NA | Spanned the incubation period | Continuous | 7.5 days | External piping | Vleck and Kenagy (1980) |
Procellariiformes | Hydrobatidae | Fork-tailed Storm-petrel | Oceanodroma furcata | Biparental incubators | Laboratory | 30 | NA | Spanned the incubation period | Continuous | 4.5 days | 20 | Vleck and Kenagy (1980) |
Procellariiformes | Hydrobatidae | Fork-tailed Storm-petrel | Oceanodroma furcata | Biparental incubators | Laboratory | 34 | NA | Spanned the incubation period | Continuous | 11.6 days | 100 | Vleck and Kenagy (1980) |
Procellariiformes | Procellariidae | Manx Shearwater | Procellaria puffinus | Uniparental incubator | Skokholm Bird Observatory, Pembrokeshire | 16.7 | 7 | Spanned the incubation period | Continuous | 2 days | > 50 | Matthews (1954) |
Procellariiformes | Procellariidae | Manx Shearwater | Procellaria puffinus | Uniparental incubator | Laboratory | 19.7 | 14 | Spanned the incubation period | Continuous | < 50 | Matthews (1954) | |
Charadriiformes | Alcidae | Cassin’s Auklets | Ptychoramphus aleuticus | Biparental incubators | Southeast farallon Island, California | NA | 7 days | Spanned the incubation period | Continuous | 7 days | 51.6 | Astheimer (1991) |
Charadriiformes | Alcidae | Black Guillemot | Cepphus grylle | Biparental incubators | Cooper Island, Alaska | 2.9 | 14 days | Spanned the incubation period | Continuous | 15 days | 33 | Divoky and Harter (2007) |
Charadriiformes | Laridae | Western Gulls | Larus occidentalis wymani | Uniparental incubator | San Nicolas Island, California, USA | 6 | NA | Spanned the incubation period | Nightly | NA | 90 | Bennett et al. (1981) |
Charadriiformes | Laridae | Heermann’s Gull | Larus heermanni | Uniparental incubator | Isla Rasa, California, USA | 7.9 | NA | Spanned the incubation period | 1 h | NA | 100 | Bennett and Dawson (1979) |
Galliformes | Phasianidae | Sichuan Partridge | Arborophila rufipectus | Uniparental incubator | Laojunshan National Nature Reserve, China | 13.2 | NA | Spanned the incubation period | 4.5 h daily | 9 days | 88.4 | Fu et al. (2017) |
Galliformes | Phasianidae | Japanese Quail | Coturnix japonica | Uniparental incubator | Laboratory | 10 | NA | Spanned the incubation period | 6 h daily | 5 days | 100 | Zhao et al. (2017) |
Galliformes | Phasianidae | Chicken | Gallus domesticus | Uniparental incubator | Laboratory | 10 | NA | Spanned the incubation period | 6 h daily | 7 days | 100 | Zhao et al. (2017) |
Galliformes | Phasianidae | Blood Pheasant | Ithaginis cruentus | Uniparental incubator | Lianhaushan Nature Reserve, China | 4.2 | Spanned the incubation period | 6.6 h | 9 days | Jia et al. (2010) | ||
Galliformes | Phasianidae | Ring-necked Pheasant | Phasianus colchicus | Uniparental incubator | Laboratory | 7.2 | NA | E22 | 12 h | NA | 11 | MacMullan and Eberhardt (1953) |
Galliformes | Phasianidae | Ring-necked Pheasant | Phasianus colchicus | Uniparental incubator | Laboratory | 0 | NA | E22 | 7 h | NA | 20 | MacMullan and Eberhardt (1953) |
Columbiformes | Columbidae | Pigeon | Columba livia domestica | Uniparental incubator | Laboratory | 10 | NA | Spanned the incubation period | 6 h daily | 5 days | 80 | Zhao et al. (2017) |
Anseriformes | Anatidae | Mallard | Anas platyrhynchos | Uniparental incubator | Delta, Manitoba | 0 | NA | E0 - E9 | 10 h | NA | 45 | Batt and Cornwell (1972) |
Anseriformes | Anatidae | Mallard | Anas platyrhynchos | Uniparental incubator | Delta, Manitoba | 0 | NA | E12 - E24 | 10 h | NA | 0 | Batt and Cornwell (1972) |
Passeriformes | Paridae | Great Tit | Parus major | Uniparental incubator | Łódź, central Poland | 2.5 | NA | Spanned the incubation period | Continuous | 4.64 days | 40 | Glądalski et al. (2020) |
Passeriformes | Turdidae | European Blackbird | Turdus merula | Uniparental incubator | University Botanic Garden, Cambridge, England | 9.1 | Nest deserted | Externally piped | 8 h | NA | 100 | Magrath (1988) |
Passeriformes | Estrildidae | Zebra Finch | Taenia guttata | Uniparental incubator | Laboratory | 5 | NA | Spanned the incubation period | 22 min per hour for 15 h each day | 2 days | 87.5 | Olson et al. (2006) |
Passeriformes | Alaudidae | Horned Lark | Eremophila alpestris | Uniparental incubator | Hudson bay mountain, British Clumbia, Canada | 6.06 | NA | Spanned the incubation period | 2 h | 2 days | 90 | MacDonald et al. (2013) |
Apodiformes | Trochilidae | Broad-tailed Humming-bird | Selasphorus platycercus | Uniparental incubator | Gothic, Gunnison County, Colorado | −1 | NA | Spanned the incubation period | Nightly | NA | 50 | Calder and Booser (1973) |
Psittaciformes | Psittaculidae | Budgerigar | Melopsittacus undulatus | Uniparental incubator | Laboratory | 10 | NA | Spanned the incubation period | 6 h daily | 4 days | 55 | Zhao et al. (2017) |
Sphenisciformes | Spheniscidae | Magellanic Penguin | Spheniscus magellanicus | Biparental incubators | Isla Quiroga, Santa Cruz Province, Argentina | NA | NA | Spanned the incubation period | 9 h | No increase | 100 | Barrionuevo and Frere (2012) |
Podicipediformes | Podicipedidae | Red-necked Grebe | Podiceps grisegina | Biparental incubators | Lake Osakis | 17 | NA | Spanned the incubation period | 3–9 h | 10 days | High | Nuechterlein and Buitron (2002) |
Falconiformes | Falconidae | American Kestrel | Falco sparverius | Uniparental incubator | Eastern Washington, USA | 12.7 | NA | E15 | 21 h | 2.5 days | 33 | Sockman and Schwabl (1998) |
In both precocial and altricial species, heart rates (HR) can be detected from about 33% into their incubation periods (Tazawa et al., 1994). In chicken embryos (a precocial species), a detectable heart rate is observed around embryonic day (E) 4–6 (Lierz et al., 2006; Sarre et al., 2006), while in altricial species such as Zebra Finch or Common Cuckoos (Cuculus canorus) embryos, it is observed around E3.5 (Coe et al., 2015; Sheldon et al., 2018). Incidentally, avian embryos during this period exhibit the highest cold tolerance (Fig. 2). As the avian embryo is developing, its HR increases while cold tolerance decreases (Tazawa et al., 1994). When ambient temperature drops rapidly, the HR of embryos also drops exponentially (Andrewartha et al., 2011; Vostarek et al., 2016). What happens is that, first, a temperature threshold is reached that initiates the drop in HR (upper threshold); if temperatures continue dropping then a threshold is reached that causes cessation of heartbeats (lower threshold), otherwise known as cardiac arrest. How avian embryos’ HR respond to drop in temperatures is species-specific. The HR of chicken embryos may start dropping from as high as 36 ℃ (1 ℃ less than the optimum) and they enter cardiac arrest at 17.9 ℃ (Mortola, 2006; Sarre et al., 2006). In temperate seabird embryos (Charadriiformes), however, HR starts dropping only after temperatures fall below 30 ℃, and they enter cardiac arrests at 11 ℃ (Western Gull Larus occidentalis; Bennett et al., 1981) or as low as 7.9 ℃ (Heermann’s Gull L. heermanni; Bennett and Dawson, 1979). Embryos may restart their cardiac activity once they are rewarmed above their lower threshold temperatures.
While modulation of HR in response to dropping temperatures is more species-specific, egg temperatures under natural incubation also differ, but the difference has to do more with breeding ecology. Under active incubation, egg temperatures of Arctic birds may be as low as 29.7 ℃; in warmer environments egg temperatures are maintatined between 32 and 34 ℃ (Boersma et al., 1980). This difference is attributed to heat loss that is more apparent in eggs from colder environments than that of eggs from warmer environments, even if incubating parents from both environments produced similar incubation temperatures. However, the mechanisms by which heart rate and metabolism affect the cold tolerance of bird embryos are not yet fully understood. Also, we are yet to fully understand how avian embryos detect and respond to severe cold temperatures before the brain, hypothalamic-pituitary-adrenal (HPA), or hypothalamic-pituitary-thyroid (HPT) axes are fully formed and functional.
In endotherms, the rate of oxygen consumption is directly proportional to metabolism. When adults are exposed to cold, they upregulate their metabolism to counter the cold stress. In avian embryos, however, the cooler the temperature, the slower the metabolism (Black and Burggren, 2004). Despite this, some metabolic processes persist until the species-specific lower threshold temperature for cardiac arrest is reached (Stoleson, 1999). This threshold temperature is consistent across embryonic age; but the extent to which avian embryos slow-down metabolism is highly dependent on the duration of cold exposure and the embryonic age (Sauve et al., 2021). Avian embryos can slow-down their oxygen consumption rate because hypothermia inhibits action potentials in the embryonic myocardium (Klein and Evans, 1961). For example, when the incubation temperatures of chicken embryos were lowered to between 30 and 20 ℃, both potassium ion influx and efflux were inhibited (active and passive movements respectively). When the temperatures were further lowered to between 10 and 5 ℃, potassium ion influx was inhibited more than its efflux (Klein and Evans, 1961). Therefore, physiological zero temperatures (24–28 ℃) are the temperatures above which incubation is initiated. However, once incubation has started, temperatures below physiological zero do not entirely suspend embryonic development.
Animals in torpor decrease or suspend metabolism, oxygen consumption rate, and cardiac activity (Schleucher, 2004). This state is observed in various avian embryos under severe cold stress, as they also suspend cardiac activity and metabolic rates. Such torpor-like states enable embryos to conserve energy, to reduce oxidative stress within the egg, but more importantly to remain alive until they detect optimum temperatures, in which they can resume normal development (Vleck and Vleck, 1996; Wentworth et al., 2009). However, this survival strategy comes at a cost, as it decelerates embryonic growth and extends the embryonic period (Table 1). While torpor is common in avian embryos, very few bird taxa retain this capability into adulthood possibly due to their high metabolism. It is will be worth investigating whether avian embryos possess similar torpor molecular mechanisms and regulatory pathways to those of true torpid animals.
The viability of avian embryos during cardiac arrest is dependent on their developmental stages and metabolism. Early-stage embryos have been observed to remain viable for more than a day while mid and late-stage embryos can only survive for a few hours (Tazawa and Rahn, 1986; Andrewartha et al., 2011). Early-stage embryos display high cold tolerance while consuming low oxygen and having shallow heartbeats (DuRant et al., 2011; Sheldon et al., 2018). In contrast, mid-incubation stage embryos are the least tolerant to cold exposure and consume the highest amount of oxygen (Suarez et al., 1996). Last-stage precocial embryos, just before hatching, exhibit increased cold tolerance compared with mid-incubation stage embryos but less than early-stage embryos (Moreng and Shaffner, 1951; Fig. 1C). Similar to early-stage embryos, last-stage precocial embryos have limited oxygen consumption and shallow heartbeats. While metabolism is positively correlated with cold tolerance in adult stages, it exhibits a negative correlation with the cold tolerance of avian embryos (Suarez et al., 1996).
Both precocial and altricial embryos exhibit different metabolic rates during the first two embryonic stages. Low metabolic rates are observed up to approximately 33% into the incubation period, while higher metabolic rates are observed up to around 62%, which is the mid-incubation period. However, precocial embryos exhibit limited metabolic rates just before hatching, which accounts for approximately 95% of the incubation period (de Oliveira et al., 2008; DuRant et al., 2011; Sheldon et al., 2018). The highest cold tolerance exhibited by early-stage embryos can be attributed to the fact that thermoregulatory setpoints have not yet been established. This suggests that tolerance to severe cold is programmed early on, before the HPT/HPA pathways are fully developed. These two axes mature during mid-incubation (de Groef et al., 2013). Interestingly, shearwater embryos display improved cold tolerance capabilities as they develop, which is not observed in other avian embryos (Procellaria puffinus; Procellariiformes; Matthews, 1954; Fig. 2A). It is currently unknown whether precocial embryos briefly improve their cold tolerance around piping stage to ensure they hatch.
The threshold temperatures that trigger cardiac arrest, for example 17.9 ℃ in chicken eggs, seems to represent the boundary of cold tolerance between early-stage embryos and more advanced embryos. This is particularly true for precocial embryos as opposed to altricial ones. As embryos mature, their thermogenesis capacity increases, and those that are exposed to temperatures above 18 ℃ during the late incubation period tend to fare better than their younger counterparts (Fig. 3C). However, embryos that are exposed to temperatures below 18 ℃ generally perform better when they are younger, likely because they can tolerate longer periods of cardiac arrest. With the exception of breeders, older chicken embryos are more cold-tolerant when exposed to temperatures above 18 ℃, whereas younger embryos exposed to temperatures below 18 ℃ exhibit greater cold tolerance (Matthews, 1954; Astheimer, 1991; Martin and Wiebe, 2004). For example, embryonic day (E) 16 chicken embryos exposed to 24 ℃ for 48 h had an 82% survival rate (Suarez et al., 1996), and E17 embryos exposed to 21.2 ℃ for 24 h had a hatching success rate of over 90% (Buckland, 1970). However, these eggs had only a 5% hatching success rate when exposed to 11.3 ℃ for 24 h, and no eggs hatched when exposed to 5.2 ℃ for 20 h. Among wild bird species, the most extreme example of mid-incubation cold tolerance was observed in penguins. The eggs of Magellanic Penguins hatch successfully, despite being left mid-incubation in the wild at temperatures as low as 6.5 ℃ for 9 h (Barrionuevo and Frere, 2012). Therefore, the temperature thresholds for embryonic development are not solely determined by phylogeny but also by life-history traits and habitat.
Interestingly, although cold-exposed embryos extend their incubation periods, they generally exhibit similar weights at hatching as embryos incubated at optimal temperatures. The reason for this phenomenon remains unclear. However, it is possible that the arrested development of cold-exposed embryos until they detect favorable temperatures may contribute to this similarity in weight. During arrested development phases, nutrient utilization is relatively low, which may prevent overall weight loss. For example, cold-treated precocial Duck (Aix sponsa) embryos and the precocial Megallanic Penguin embryos in the wild under cold conditions did not exhibit significant differences in hatchling weight or embryonic viability to their controls (DuRant et al., 2011; Barrionuevo and Frere, 2012). However, in the case of altricial Zebra Finches (Taeniopygia guttata), cold-treated embryos had reduced hatchling weights (Olson et al., 2006).
The dissimilarity in hatching weight between precocial and altricial species may be attributed to their distinct developmental trajectories. Precocial avian eggs have a higher yolk content, which serves as an energy reserve, compared with altricial birds. In cold conditions, lipids from the yolk are transferred to embryonic tissues (Olson et al., 2006). Precocial avian embryos can control heat loss through vasoconstriction by mid-incubation and thermogenic mechanisms begin towards the hatching stage (de Groef et al., 2013; Price and Dzialowski, 2017). Thus, the metabolism of cold-exposed precocial avian embryos does not decrease significantly during incubation (Astheimer, 1991; Nichelmann and Tzschentke, 2002; Gräns and Altimiras, 2007). In contrast, altricial birds develop thermogenic mechanisms 2–3 weeks post-hatching (Tazawa et al., 2001), which may explain why most cold-treated precocial embryos do not lose weight, unlike cold-treated altricial embryos. Further studies are required to investigate the similarities and differences in hatching weight, particularly at very low temperatures across diverse bird species.
It has been observed that exposure to cold temperatures prolongs the incubation period for avian embryos (as shown in Table 1). This is due to the fact that avian embryos tolerate cold conditions by slowing down or even suspending their growth and development. This process involves a reduction in the uptake of glucose or yolk lipid, depending on the stage of development—in short, the embryos downregulate their metabolic activity (Feast et al., 1998). As metabolism is responsible for driving all biological processes, a decrease in metabolic rate (resulting in reduced oxygen consumption by the egg) can partly explain the extended incubation period (Mueller et al., 2022). When exposed to cold stress, avian embryos utilize adaptive mechanisms to downregulate metabolic activity and halt active cell division, potentially arresting mitosis at the mitotic G2 phase (Rafferty and Reina, 2012; Ko et al., 2017). Surviving periodic egg neglect requires the ability to tolerate low ambient temperatures and resume normal development upon rewarming (Astheimer, 1991). To compensate for the inconvenience of cold exposure and to complete embryonic develpment, avian embryos lengthen their incubation periods, which is influenced by various factors such as phylogeny, egg size, embryonic developmental stage, ambient and incubation temperatures, and duration of neglect (Webb, 1987; Suarez et al., 1996).
In certain circumstances, the exposure of eggs to cold and the subsequent extension of the incubation period result in negative consequences of varying degrees (Boersma et al., 1980). These negative outcomes can include: abnormal embryonic growth, hindrance in the development of hatching muscles, and mortality due to inefficiency in nutrient uptake, below the level required for embryo survival. Mortality may also occur in embryos that had struggled to reach the piping stage but are incaple of hatching, because they are physically and nutritionally exhausted. Mortality may be lower for some lucky eggs that reach piping stage under natural incubation: they may be assisted either by the incubating parents or by earlier hatched chicks in highly precocial species. However, parental or sibling assistance during hatching is not typically observed in laboratory experiments, which may partly explain why hatching rates in the laboratory and the wild may differ.
Avian embryos exposed to cold stress compensate for delayed growth during the extended incubation period. The compensatory growth is a cumulative process. Different durations of cold exposure during disproportionate embryonic days are compensated for cumulatively (Table 1; Feast et al., 1998). This compensation occurs even when more than 80% of the incubation period is below optimal temperatures (Dawson, 1984). Hence, in a given species, the cumulative periods of cold exposure or egg neglect are directly proportional to the increase in the incubation period (Boersma and Wheelwright, 1979; Astheimer, 1991; Mortola, 2006). Compensatory growth (reviewed in Webb, 1987) occurs even when the growing embryos experience severe cold stress throughout their incubation periods (Barrionuevo and Frere, 2012; Zhao et al., 2017). While cold temperatures cause embryonic development to be suspended extensively, high temperatures accelerate embryonic development and shorten the incubation period (Griffith et al., 2016). However, the mechanisms used by bird embryos to control cumulative compensatory growth are still not fully understood.
Numerous factors, both intrinsic and extrinsic, influence the cold tolerance of avian embryos (as depicted in Fig. 4). Certain factors are significant prior to the onset of incubation, while others gain greater importance during incubation, and still others remain important throughout the entire period leading up to hatching. These factors are not mutually exclusive and can interact in complex ways.
During the pre-breeding stage, birds exhibit a discerning approach to selecting nesting sites based on several factors, including local climatic conditions, availability of nesting materials, and food resources (Stillman et al., 2019). These choices are aimed at providing the ideal microclimate for embryonic development (Heenan et al., 2015; Perez et al., 2020). For example, breeding birds in high-latitude or high-altitude regions construct nests with higher heat retention properties than their counterparts in temperate and tropical regions. High-latitude summer breeders tend to opt for more compact nests or crevices that maximize heat retention and insulation, while tropical breeders make more porous nests using materials with high thermal conductivity to facilitate quick heat loss (Heenan et al., 2015). Such nesting site selection strategies are essential for the fitness of embryos.
Certain bird species, particularly those inhabiting aquatic environments, make use of burrows as their nesting sites. However, these burrow nests benefit tropical aquatic birds more than their Arctic counterparts. For instance, the burrow nest of the Crab Plover (Dromas ardeola), a tropical shorebird, offers optimal incubation conditions—35.2 ℃ and a relative humidity of 60.2%—allowing for low incubation attendance (de Marchi et al., 2008). On the other hand, the nest cavity of the Black Guillemot, an Arctic seabird, can drop to a daily mean temperature of 2.9 ℃ (Divoky and Harter, 2007). Therefore, egg neglect is not a significant selective pressure that hinders embryonic viability among most tropical birds. However, embryos from cold climates must adapt to long egg neglect under extremely cold conditions and develop greater tolerance to cold stress to survive.
Clutch size is a crucial factor that affects the nest microclimate during embryonic development. In stable temperatures, eggs belonging to large clutches, particularly those located at the bottom or the center, retain and share more heat; while eggs from small clutches lose heat more easily (Calder and Booser, 1973). In fluctuating temperatures, however, eggs located at the upper levels of the clutch (typically later-laid eggs) tend to develop faster than those at lower levels levels (Huo et al., 2018; Duan et al., 2019). Nevertheless, a large clutch requires greater nest attentiveness and a longer incubation period. This is because incubating parents cannot ensure optimum incubation temperatures for all eggs at all times, leading to hatching asynchrony—some eggs hatching earlier than others—thus prolonging nest residency (a situation that endangers both the parents and the hatclings as they become more vulnerable to predation) (Stoleson and Beissinger, 1995).
Furthermore, early-laid eggs from large clutches may experience longer egg neglects, especially for species that do not start incubation until the clutch is complete (Wang and Beissinger, 2011). Such prolonged incubation periods may negatively affect fitness due to increased predation risk and nest abandonment. This may explain why most Arctic and seabirds tend to have smaller clutches. Despite having a smaller clutch, seagulls, such as the Ring-billed Gull (Larus delawarensis), may abandon other pipped eggs after the first egg hatches (Evans, 1990). Thus, birds breeding in colder environments may face a tradeoff between investing in a larger clutch and incurring longer nest residency or in a small clutch with lower hatching success.
The avian egg is a self-contained oval entity comprising an outer eggshell that serves as a protective barrier against physical harm and microbial contamination, regulates gaseous and water exchange, and provides calcium to the developing embryo (Hincke et al., 2011). Eggshells are the first protective barrier against cold temperatures. Thus, eggs having lower shell conductance require longer incubation periods (Mueller et al., 2022). The internal environment of the egg consists of albumen (or egg white), and egg yolk, which contains the embryo and the bulk of the its nourishment (Vieira, 2007; van der Wagt et al., 2020). The yolk is rich in water, lipids, proteins, carbohydrates, and minerals, which are necessary for the developing embryo (Anton, 2007, 2013). Yolk lipids account for 90% of the energy required by the embryo (van der Wagt et al., 2020). During incubation, the egg loses approximately 75% of its weight due to evaporative water loss. Because egg neglect is inevitable for avian embryos, certain egg structures have evolved to minimize the effect of the neglect. For example, the air spaces of neglected eggs cool faster than the rest of the egg, reducing water loss by about 50% (Simkiss, 1974).
The quality of eggs laid by females is influenced by their nutritional and physical well-being, which in turn affects the amount of egg yolk and hormones deposited (de Groef et al., 2013). The level of maternal investment in eggs is a crucial factor in determining the ability of an embryo to tolerate stressful conditions such as cold temperatures. Eggs of lower quality have longer incubation periods, indicating slower embryo development compared with those from higher quality eggs. As a result, the combination of low egg quality and cold environmental temperatures will be detrimental on the fitness of growing avian embryos.
The structural and nutritional readiness of the embryo to cope with cold stress is directly proportional to the quality of the egg. Therefore, the composition of the egg, including the quantity of macronutrients and micronutrients, plays a critical role. It is apparent that the quality of eggs is solely dependent on the mother hen (Hill, 1993; Nelson et al., 2010; Widowski et al., 2022). Inherent factors of the mother hen that determine the egg quality are: her maternal environment (Widowski et al., 2022), age (Hulet et al., 2007; Cherian, 2008; Yalçin et al., 2008; Nonis and Gous, 2013), genotype (Sirri et al., 2018; Duan et al., 2019; Werner et al., 2019; Nishimura et al., 2021), nutritional status, quality of drinking water, and body condition index (Roberts, 2004; Nonis and Gous, 2013; Filipiak-Florkiewicz et al., 2017; Kowalska et al., 2021; Widowski et al., 2022).
Of all the aforementioned factors of the laying mother, nutritional status, age, and genotype, are the most paramount. These factors play crucial roles in determining how developing embryos respond, adapt and survive to cold stress. Eggshell quality is primarily affected by the level of stress (e.g., heat, cold, and population density); while egg internal quality is more influenced by other factors such as storage conditions (like duration; Reijrink et al., 2010), temperature, type of feed, feed enzymes, medication, and hypoxia (Roberts, 2004; Jia et al., 2016).
We may think that small eggs may be more vulnerable to cold exposure due to their higher thermal inertia than larger eggs (Batt and Cornwell, 1972), and because larger eggs contain more energy content and insulation (Mueller et al., 2022). However, the effect of egg size on the cold tolerance of avian embryos is generally minimal. For instance, storm petrel eggs, with an average size of 12.5 g, can hatch after they are neglected for 15 days at a nest temperature of 10 ℃ (Boersma and Wheelwright, 1979). In a study involving eggs of various sizes—ranging from 2.6 to 51.6 g—from four domestic birds, namely Budgerigar (Melopsittacus undulatus), Japanese Quail, Pigeon (Columba livia), and chickens, more than 60% of the eggs hatched after experiencing a daily 10 ℃ cold for 6 h throughout their incubation periods (Zhao et al., 2017). The question of the minimum egg size that cannot survive extremely cold temperatures arises. Calder and Booser (1973) observed that the eggs of Broad-tailed Hummingbirds (Selasphorus platycercus), which weigh approximately 1.4 g, can survive cold nights (−1 ℃) after their internal egg temperatures drop to 6.5 ℃. Theoretically, the egg size that cannot tolerate chilling temperatures may be below that of hummingbirds. Embryos of White-rumped Munia (Lonchura striata), with an egg size of 1.07 g, died after 10 days under a daily 10 ℃ cold for 6 h (Zhao et al., 2017). The observed survival of munia embryos within the first 10 days, despite the size of their eggs, suggests that they might have hatched if the duration or severity of the cold exposure was reduced. In birds, the effect of egg size becomes more pronounced post-hatching, where it is more associated with chick survival (Boersma et al., 1980).
The colors present on the surface of eggs have a crucial role in regulating the temperature of embryos. Pigments can affect the ability of eggs to retain and absorb heat, which is important for the survival of embryos. In hot climates, eggs laid in exposed nests have a higher reflectivity due to the presence of whiter pigmentation (Kilner, 2006). In contrast, eggs laid in colder climates have more pigments that absorb near-infrared wavelengths, which leads to higher eggshell absorbance (Liao, 2021). The laying sequence of eggs can also affect eggshell reflectance. Later laid eggs of Russet Sparrow (Passer cinnamomeus) had higher reflectivity, and they developed faster, indicating that they absorbed more incubation warmth than earlier laid eggs (Huo et al., 2018). A global analysis has shown that eggshells from colder habitats are darker, as dark surfaces warm up faster than lighter surfaces upon exposure to incident solar radiation (Wisocki et al., 2020). Furthermore, eggs of birds that breed in cold climates have low thermal emissivity (Björn et al., 2016). It would be interesting to investigate the types of pigments present in eggs of birds that breed in colder environments or seasons and how these pigments contribute to cold tolerance.
Interestingly, whether precocial birds breed in warmer or colder environments, they lay eggs with lower thermal emmisivity than altricial eggs. The higher thermal emissivity of the altricial eggs, is partly due to the fragility of their egg cuticle (Björn et al., 2016). In altricial species, Passeriformes eggs display a greater thermal emissivity relative to non-Passeriformes. For example, Calidris spp. that breed in the tundra with uniparental nest attentiveness, have lower eggshell thermal emissivity than that of passerines (Björn et al., 2016). Nonetheless, it is yet to be determined whether the low thermal emissivity in the eggs of Calidris genus members arose from evolutionary pressure to mitigate the effects of egg size, fragility, and low parental attendance.
During the incubation period, bird parents face the challenge of balancing the energy demands of keeping their eggs warm and maintaining their own body temperature in low ambient temperatures. This creates a trade-off between foraging for food to meet their nutritional and thermoregulatory needs during the day and ensuring continuous warmth for their eggs. When facing adverse conditions, parents prioritize self-maintenance over nest attentiveness, which can result in reduced care or even abandonment of the eggs, increasing the risk for the embryos. Thus, egg neglect is common during food shortages, which often occur during cold breeding seasons. To mitigate this challenge, breeding birds lay their eggs during seasons of food abundance. However, this is not always feasible for Arctic and sea birds.
Cold environments can have an adverse effect on breeding birds, leading to a suppression of their immune system and making them more susceptible to infection (Cheville, 1979). A study conducted on Great Tits revealed that they increased their mean plasma hemoglobin concentration, an indicator of body condition, following a sudden drop in temperature in Europe between 2017 and 2018 (Glądalski et al., 2020). Apart from cold environments, factors such as low food availability, which may result from sparse or distant foraging grounds (Boersma and Wheelwright, 1979), predation (Nuechterlein and Buitron, 2002), and human-induced nest disturbance (Macdonald et al., 2013), also affect the body condition of breeding birds. As a result, these challenges in maintaining body condition can reduce nest attentiveness, which can have a negative effect on the growth and development of avian embryos.
Birds possess a unique characteristic among the animal kingdom. They physically incubate their eggs until they hatch. This is facilitated by an increase in prolactin levels after egg-laying, guaranteeing strong parental commitment (Lormée et al., 1999). Incubating birds often develop a highly vascularized ventral bare skin, commonly referred to as a “brood patch”, which aids in the incubation process: it warms cooled eggs and cools over-heated eggs (Dawson, 1984). When the brood patch comes into contact with cooled eggs, thermoreceptors located both peripherally and centrally stimulate heartbeat, shivering (Tøien, 1993), and likely non-shivering thermogenesis to increase (Bicudo et al., 2002; Nowack et al., 2017). The stimulation of thermogenesis becomes stronger as eggs get cooler. When they detect sub-optimal temperatures, late-stage avian embryos alert their neglecting parents by begging for more nest attentiveness through emitting “distress calls” or increasing their motility (Gräns and Altimiras, 2007; Mortola, 2019).
Breeding birds living in cold environments have developed a strategy to increase their cold tolerance by increasing their summit and maximum metabolic rates through shivering, depending on their size (Swanson and Vézina, 2015). The increase in metabolic rates also benefits the dependent embryos within the eggs, as it ensures increased warmth and a guarantee of not being abandoned by the parents (Martin and Wiebe, 2004). When eggshell temperatures decrease from the optimum until 15 ℃, parents can increase their basal metabolic rates up to four-fold to rewarm their eggs. However, below this temperature, the metabolic rate plateaus, indicating that the maximum metabolic rate has been reached (Tøien, 1993). Hence, avian embryos in very cold nest microclimates, where eggshell temperatures frequently fall below 15 ℃, are put to the test in terms of their cold tolerance (Divoky and Harter, 2007).
The physiological process of circadian rhythm results in a decrease in body temperature, including that of incubating parents, during the night (Refinetti, 2016). Nonetheless, some seabirds only incubate their eggs during the night due to low food availability or fear of predators (Martin and Wiebe, 2004). This means that their embryos get incubation warmth only at night. However, night incubation may not result in much warmth due to circadian rhythm and because night incubation does not easily elicit surplus metabolic rates for greater heat production. For example, incubating Flycatchers (Empidonaox oberholseri) only initiate shivering thermogenesis at night when the ambient temperature drops to 4 ℃ (Morton and Pereyra, 1985). As a result, eggs under active incubation may become too cold at night, leading to cardiac arrest; nevertheless, they can recover quickly if re-warmed (Bennett and Dawson, 1979; Bennett et al., 1981). The incubating parents face costly trade-offs, particularly when exposed to prolonged cold ambient temperatures and long incubation periods, which increase thermogenic demands. In addition, food shortages compromise the body condition of the incubating parents, resulting in longer egg neglects (Macdonald et al., 2013; Coe et al., 2015) and, in extreme cases, nest abandonment, leading to embryonic mortality (Boersma et al., 1980).
Three distinct types of incubation behaviors exist among birds, namely biparental, uniparental, and partial incubation, depending on the level of investment made by the parents. In biparental incubation, both parents take turns to incubate the eggs to minimize offbout durations (Groscolas and Robin, 2001). This approach offers both parents ample opportunities for rest and foraging, while simultaneously minimizing the risk of the eggs cooling due to neglect (Cantar and Montgomerie, 1985; Auer et al., 2007; Brynychová et al., 2020). Despite being a mutually beneficial approach, biparental incubation is less common in birds (Auer et al., 2007); after incubation however, biparental care becomes more common as it is observed in over 90% of bird species (Wesolowski, 1994). On the other hand, uniparental incubation is the most prevalent incubation behavior among birds, even though it is more demanding for the incubating parent and their embryos, particularly in colder regions.
Uniparental incubators face a significant challenge in balancing their time between foraging and incubation (Jia et al., 2010; Fu et al., 2017). While parents need to take breaks to forage, this leaves their eggs unattended, which can be detrimental to the development of their ectothermic embryos. In cold climates, uniparental incubators such as Sichuan Partridges and Blood Pheasants have adapted to reduce egg neglect by taking longer, single off-bouts daily (Jia et al., 2010; Fu et al., 2017). Still, Arctic and temperate avian embryos with uniparental incubators experience longer periods of egg neglect (Tulp and Schekkerman, 2006). To address this challenge, some male birds have developed a strategy of foraging for their incubating female partners (Martin and Wiebe, 2004; Rohwer and Purcell, 2019). This provisioning behavior reduces the duration of egg neglect and exposure to very cold temperatures.
Partial incubation is a behavior whereby eggs that have been laid earlier are intermittently incubated before the entire clutch is completed. This behavior may increase hatchability (Batt and Cornwell, 1972; Stoleson, 1999; Wang and Beissinger, 2011). In some cases, however, partial incubation primarily provides warmth to the eggs, thereby minimizing egg cold exposure, rather than proper incubation (Morton and Pereyra, 1985). For example, in birds such as flycatchers, egg temperatures may remain below their physiological zero temperatures 83% of the time during partial incubation (Morton and Pereyra, 1985). Thus, it has been argued since the 1940s that this behavior should, instead, be reffered to as “brooding” (Ryves, 1946).
Despite the advantages of partial incubation, unincubated eggs tolerate longer cold exposures than eggs undergoing incubation (Batt and Cornwell, 1972). This is due to the fact that heat triggers irreversible physiological processes and increases embryos’ sensitivity to temperature fluctuations (Stoleson, 1999). Therefore, the likelihood of partial incubation decreases in cold climates, particularly among altricial passerines like House Wrens (Troglodytes aedon; Schaming, 2010). In some species, such as Great Tits and Blue Tits (Cyanistes caeruleus), incubation can be postponed even after the clutch is completed (Whitehouse et al., 2013). This delay not only benefits the embryos, but also allows parents to forage for longer periods to increase energy storage and ensure self-maintenance.
There are differences in the cold tolerance of avian embryos depending on whether they are precocial or altricial. Precocial bird eggs contain more yolk than altricial eggs, which results in precocial embryos generating more heat, having more insulation, and having a stronger ability to regulate their body temperature. Precocial embryos have evolved to initiate heat loss control and thermogenesis earlier than altricial embryos (Tazawa et al., 2001; De Groef et al., 2013; Price and Dzialowski, 2017). The cold tolerance of birds is determined by their phylogeny, specifically whether they exhibit acute or chronic cold tolerance, rather than the severity of the cold stress. Wild birds that breed in polar or highland regions, such as Procellariiformes, Charadriiformes, and Sphenisciformes, exhibit greater chronic cold tolerance. Although some Anseriformes and Galliformes sympatrically coexist with Procellariiformes, their embryos can only survive acute cold exposure for one day.
The phenomenon of cold tolerance in artificially selected bird species, such as Domestic Chickens, is intriguing. Despite being subjected to over 8000 years of selective breeding and domestication under favorable conditions (Hata et al., 2021), these birds continue to exhibit cold tolerance. This indicates that the heritable cold tolerance trait is more closely linked to phylogeny rather than breeding ecology. Numerous studies conducted on commercial broilers have also produced comparable results, suggesting that this cold tolerance capability has been retained even after centuries of crossbreeding and domestication. However, it is important to exercise caution when extrapolating experimental results to natural settings. Although the conditions may be similar, the outcomes of domesticated birds tend to differ from those of their wild counterparts, achieving higher levels of success on average, as evidenced by research on experimental incubation (Ospina et al., 2018).
Avian embryos of all species, whether wild, domesticated, native, or hybrid, possess the ability to tolerate severe cold temperatures. The capacity of avian embryos to tolerate cold conditions is influenced by both intrinsic and extrinsic factors, in addition to their phylogenetic background. When exposed to cold temperatures, avian embryos reduce their heart rates and metabolism, eventually entering a torpid-like state of cardiac arrest. The duration of this torpid-like state depends on the developmental stage of the embryo, with those in early incubation and piping stages exhibiting greater cold tolerance than those in mid-incubation. Cold conditions during incubation result in a cumulative compensatory growth of the embryos, leading to an extended incubation period. However, some species may experience reduced hatching weight due to cold conditions during incubation. The cold tolerance of embryos is also influenced by several extrinsic factors, including nest architecture, clutch size, egg characteristics before incubation, and parental behaviors after the start of incubation.
Despite the potential negative effects of severe cold exposure on avian embryos, they have been observed to possess a certain level of adaptability to inclement weather conditions and climate variability. This adaptability suggests that avian embryos are capable of adjusting to cooler climates, with the exception of global warming. However, there is currently a lack of data on the tolerance of various bird species to extremely low temperatures. While studies on the mild cold tolerance of domestic birds are prevalent, research on the cold tolerance of diverse species from different orders residing in both cold and warm climates is necessary to fully comprehend the under-reported resilience of avian embryos. Specifically, further investigation is required to understand how avian embryos regulate their heart rate and metabolism during different embryonic stages, as well as the underlying mechanisms that enable these embryos to detect external signals and modulate their molecular and physiological pathways. It is imperative that additional research is conducted to address these fundamental questions.
DL and IMA conceived the idea, collected the data, and wrote the final draft. Both authors read and approved the final manuscript.
The authors declare that they have no competing interests.
This work was supported by the National Natural Science Foundation of China (NSFC, 31971413) granted to DL and the Second Tibetan Plateau Scientific Expedition and Research Program (STEP, 2019QZKK0501) granted to DL.
1. | Qingmiao Yuan, Xi Lu, Ruixin Mo, et al. Development and Parentage Analysis of SNP Markers for Chestnut-Vented Nuthatch (Sitta nagaensis) based on ddRAD-seq Data. Avian Research, 2024. DOI:10.1016/j.avrs.2024.100179 |
2. | Huan Liu, Yun Fang, Yingqiang Lou, et al. A high level of extra-pair paternity in the Chestnut Thrush (Turdus rubrocanus). Avian Research, 2023, 14: 100135. DOI:10.1016/j.avrs.2023.100135 |
Order | Family | Common name | Scientific name | Incubation behavior | Breeding locality | Ambient/experimental temperatures (℃) | Days of egg neglect | Embryonic age of exposure | Duration of cold exposure | Increase in incubation period | Hatching success (%) | References |
Procellariiformes | Hydrobatidae | Fork-tailed Storm-petrel | Oceanodroma furcata | Biparental incubators | East Amatuli Island, Barren Islands, Alaska, USA | 10 | 7.6 days | Spanned the incubation period | Continuous | 9.3 days | 100 | Boersma and Wheelwright (1979) |
Procellariiformes | Hydrobatidae | Fork-tailed Storm-petrel | Oceanodroma furcata | Biparental incubators | East Amatuli Island, Barren Islands, Alaska, USA | 10 | 15.5 days | Spanned the incubation period | Continuous | 17.6 days | 100 | Boersma and Wheelwright (1979) |
Procellariiformes | Hydrobatidae | Fork-tailed Storm-petrel | Oceanodroma furcata | Biparental incubators | East Amatuli Island, Barren Islands, Alaska, USA | 10 | 28 days | Spanned the incubation period | Continuous | 23 days | 100 | Boersma et al. (1980) |
Procellariiformes | Hydrobatidae | Fork-tailed Storm-petrel | Oceanodroma furcata | Biparental incubators | Laboratory | 26 | NA | Spanned the incubation period | Continuous | 7.5 days | External piping | Vleck and Kenagy (1980) |
Procellariiformes | Hydrobatidae | Fork-tailed Storm-petrel | Oceanodroma furcata | Biparental incubators | Laboratory | 30 | NA | Spanned the incubation period | Continuous | 4.5 days | 20 | Vleck and Kenagy (1980) |
Procellariiformes | Hydrobatidae | Fork-tailed Storm-petrel | Oceanodroma furcata | Biparental incubators | Laboratory | 34 | NA | Spanned the incubation period | Continuous | 11.6 days | 100 | Vleck and Kenagy (1980) |
Procellariiformes | Procellariidae | Manx Shearwater | Procellaria puffinus | Uniparental incubator | Skokholm Bird Observatory, Pembrokeshire | 16.7 | 7 | Spanned the incubation period | Continuous | 2 days | > 50 | Matthews (1954) |
Procellariiformes | Procellariidae | Manx Shearwater | Procellaria puffinus | Uniparental incubator | Laboratory | 19.7 | 14 | Spanned the incubation period | Continuous | < 50 | Matthews (1954) | |
Charadriiformes | Alcidae | Cassin’s Auklets | Ptychoramphus aleuticus | Biparental incubators | Southeast farallon Island, California | NA | 7 days | Spanned the incubation period | Continuous | 7 days | 51.6 | Astheimer (1991) |
Charadriiformes | Alcidae | Black Guillemot | Cepphus grylle | Biparental incubators | Cooper Island, Alaska | 2.9 | 14 days | Spanned the incubation period | Continuous | 15 days | 33 | Divoky and Harter (2007) |
Charadriiformes | Laridae | Western Gulls | Larus occidentalis wymani | Uniparental incubator | San Nicolas Island, California, USA | 6 | NA | Spanned the incubation period | Nightly | NA | 90 | Bennett et al. (1981) |
Charadriiformes | Laridae | Heermann’s Gull | Larus heermanni | Uniparental incubator | Isla Rasa, California, USA | 7.9 | NA | Spanned the incubation period | 1 h | NA | 100 | Bennett and Dawson (1979) |
Galliformes | Phasianidae | Sichuan Partridge | Arborophila rufipectus | Uniparental incubator | Laojunshan National Nature Reserve, China | 13.2 | NA | Spanned the incubation period | 4.5 h daily | 9 days | 88.4 | Fu et al. (2017) |
Galliformes | Phasianidae | Japanese Quail | Coturnix japonica | Uniparental incubator | Laboratory | 10 | NA | Spanned the incubation period | 6 h daily | 5 days | 100 | Zhao et al. (2017) |
Galliformes | Phasianidae | Chicken | Gallus domesticus | Uniparental incubator | Laboratory | 10 | NA | Spanned the incubation period | 6 h daily | 7 days | 100 | Zhao et al. (2017) |
Galliformes | Phasianidae | Blood Pheasant | Ithaginis cruentus | Uniparental incubator | Lianhaushan Nature Reserve, China | 4.2 | Spanned the incubation period | 6.6 h | 9 days | Jia et al. (2010) | ||
Galliformes | Phasianidae | Ring-necked Pheasant | Phasianus colchicus | Uniparental incubator | Laboratory | 7.2 | NA | E22 | 12 h | NA | 11 | MacMullan and Eberhardt (1953) |
Galliformes | Phasianidae | Ring-necked Pheasant | Phasianus colchicus | Uniparental incubator | Laboratory | 0 | NA | E22 | 7 h | NA | 20 | MacMullan and Eberhardt (1953) |
Columbiformes | Columbidae | Pigeon | Columba livia domestica | Uniparental incubator | Laboratory | 10 | NA | Spanned the incubation period | 6 h daily | 5 days | 80 | Zhao et al. (2017) |
Anseriformes | Anatidae | Mallard | Anas platyrhynchos | Uniparental incubator | Delta, Manitoba | 0 | NA | E0 - E9 | 10 h | NA | 45 | Batt and Cornwell (1972) |
Anseriformes | Anatidae | Mallard | Anas platyrhynchos | Uniparental incubator | Delta, Manitoba | 0 | NA | E12 - E24 | 10 h | NA | 0 | Batt and Cornwell (1972) |
Passeriformes | Paridae | Great Tit | Parus major | Uniparental incubator | Łódź, central Poland | 2.5 | NA | Spanned the incubation period | Continuous | 4.64 days | 40 | Glądalski et al. (2020) |
Passeriformes | Turdidae | European Blackbird | Turdus merula | Uniparental incubator | University Botanic Garden, Cambridge, England | 9.1 | Nest deserted | Externally piped | 8 h | NA | 100 | Magrath (1988) |
Passeriformes | Estrildidae | Zebra Finch | Taenia guttata | Uniparental incubator | Laboratory | 5 | NA | Spanned the incubation period | 22 min per hour for 15 h each day | 2 days | 87.5 | Olson et al. (2006) |
Passeriformes | Alaudidae | Horned Lark | Eremophila alpestris | Uniparental incubator | Hudson bay mountain, British Clumbia, Canada | 6.06 | NA | Spanned the incubation period | 2 h | 2 days | 90 | MacDonald et al. (2013) |
Apodiformes | Trochilidae | Broad-tailed Humming-bird | Selasphorus platycercus | Uniparental incubator | Gothic, Gunnison County, Colorado | −1 | NA | Spanned the incubation period | Nightly | NA | 50 | Calder and Booser (1973) |
Psittaciformes | Psittaculidae | Budgerigar | Melopsittacus undulatus | Uniparental incubator | Laboratory | 10 | NA | Spanned the incubation period | 6 h daily | 4 days | 55 | Zhao et al. (2017) |
Sphenisciformes | Spheniscidae | Magellanic Penguin | Spheniscus magellanicus | Biparental incubators | Isla Quiroga, Santa Cruz Province, Argentina | NA | NA | Spanned the incubation period | 9 h | No increase | 100 | Barrionuevo and Frere (2012) |
Podicipediformes | Podicipedidae | Red-necked Grebe | Podiceps grisegina | Biparental incubators | Lake Osakis | 17 | NA | Spanned the incubation period | 3–9 h | 10 days | High | Nuechterlein and Buitron (2002) |
Falconiformes | Falconidae | American Kestrel | Falco sparverius | Uniparental incubator | Eastern Washington, USA | 12.7 | NA | E15 | 21 h | 2.5 days | 33 | Sockman and Schwabl (1998) |