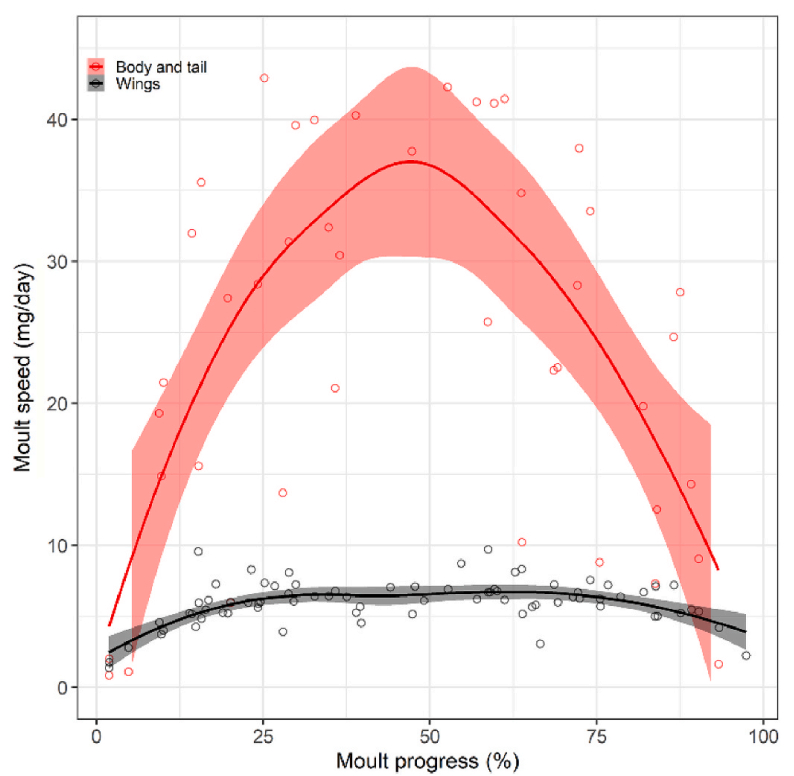
Citation: | Santi Guallar, Javier Quesada. 2023: Corrigendum to “Moult intensity constraints along the complete moult sequence of the House Sparrow (Passer domesticus)” [Avian Res. 14 (2023) 100125]. Avian Research, 14(1): 100143. DOI: 10.1016/j.avrs.2023.100143 |
In the original version of this article, we published a figure showing a gap in the confidence intervals for body and tail due to data paucity for mid stages of moult progress. Here, we amended this problem adding data from the 2023 moulting season, during which we obtained 139 moult records from 98 individuals. The final sample size used for plotting these results are shown in the caption below. This amendment corroborates the conclusion already stated: body moult does not seem to be under physiological constraints, although primary moult appears to be tightly controlled to reduce aerodynamic losses.
The authors would like to apologise for any inconvenience caused.
Santi Guallar: Conceptualization, Data curation, Formal analysis, Investigation, Methodology, Software, Validation, Visualization, Writing – original draft, Writing – review & editing. Javier Quesada: Funding acquisition, Resources, Validation, Visualization, Writing – review & editing.