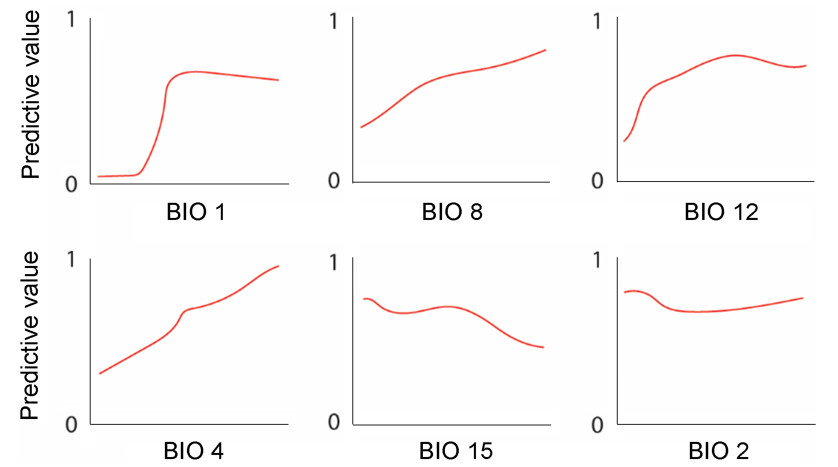
Citation: | Yashuai Zhang, Fang Wang, Zhenxia Cui, Min Li, Xia Li, Xinping Ye, Xiaoping Yu. 2021: Can we reestablish a self-sustaining population? A case study on reintroduced Crested Ibis with population viability analysis. Avian Research, 12(1): 14. DOI: 10.1186/s40657-021-00250-z |
One of the most challenging tasks in wildlife conservation and management is clarifying which and how external and intrinsic factors influence wildlife demography and long-term viability. The wild population of the Crested Ibis (Nipponia nippon) has recovered to approximately 4400, and several reintroduction programs have been carried out in China, Japan and Korea. Population viability analysis on this endangered species has been limited to the wild population, showing that the long-term population growth is restricted by the carrying capacity and inbreeding. However, gaps in knowledge of the viability of the reintroduced population and its drivers in the release environment impede the identification of the most effective population-level priorities for aiding in species recovery.
The field monitoring data were collected from a reintroduced Crested Ibis population in Ningshan, China from 2007 to 2018. An individual-based VORTEX model (Version 10.3.5.0) was used to predict the future viability of the reintroduced population by incorporating adaptive patterns of ibis movement in relation to catastrophe frequency, mortality and sex ratio.
The reintroduced population in Ningshan County is unlikely to go extinct in the next 50 years. The population size was estimated to be 367, and the population genetic diversity was estimated to be 0.97. Sensitivity analysis showed that population size and extinction probability were dependent on the carrying capacity and sex ratio. The carrying capacity is the main factor accounting for the population size and genetic diversity, while the sex ratio is the primary factor responsible for the population growth trend.
A viable population of the Crested Ibis can be established according to population viability analysis. Based on our results, conservation management should prioritize a balanced sex ratio, high-quality habitat and low mortality.
Throughout the Quaternary (the last 2.59 million years of Earth history) large continental ice sheets in the Northern Hemisphere have repeatedly advanced and retreated; these geologic processes are known as glacial and interglacial periods, respectively (Fairbridge 1972). The last 430 thousand years have been dominated by 100-thousand year glacial-interglacial cycles. Interglacial periods make up a minority (20%) of these cycles, lasting on average~10 to 30 thousand years (Philander 2008). In general, glacial periods were colder and drier than interglacial periods (Jansen et al. 2007). Glacial-interglacial cycles and their strong attendant climatic fluctuations forced species to either move to locations that remained suitable or to adapt to changing environmental conditions. The alternative outcome was extinction (Jansson and Dynesius 2002).
The most conspicuous and best documented responses of warm, temperate, and cold temperature-adapted species to these climatic fluctuations are latitudinal and altitudinal range shifts. Since the Last Glacial Maximum, warming temperatures have caused temperate and montane species to shift their ranges towards higher (northern) latitudes and altitudes (Bennett and Provan 2008; Stewart et al. 2010). These range shifts have left signals of high genetic diversity in refugia (for a detailed discussion of the refugium concept, see Stewart et al. 2010) and low genetic diversity in regions recently colonized from refugia (Hewitt 2000). Ongoing climate change is currently causing shifts in the geographic distributions of species globally and may have increased the vulnerability of some species due to rapid changes in ecological conditions (Root et al. 2003; Parmesan 2006). Understanding how species have responded to strong climatic fluctuations accompanying glacial-interglacial cycles is critical to predicting their likely future responses to climate change and can help guide conservation strategies for negatively affected species.
Predicting future distributions relies upon the use of climate change models which vary in starting assumptions (Dunn and Møller 2019), but clear scenarios of likely anthropogenic change are provided by the Intergovernmental Panel on Climate Change (IPCC 2019). Known as Representative Concentration Pathway scenarios, these can be applied to Species Distribution Models such as MaxEnt to help explore the possible range of effects of climate change on the future range of species of interest, which considers the spatial and environmental characteristics of regions of interest (Elith et al. 2011). Such modelling is the basis of predicting shifting species distribution patterns (Huntley 2019) as the world warms, and typically is used to model the effects of climate change on rare species of conservation interest or those with economic impact. More common species have been studied as well (Hitch and Leberg 2007); Common Grackle is of particular interest; it is wide-ranging, and its population and range expansion has been influenced greatly by human habitat modifications.
The Common Grackle (Quiscalus quiscula) is one of the most widespread and common migrating bird species in North America (Peer and Bollinger 1997a; Jaramillo and Burke 1999). Morphological differences indicate two well defined, and one equivocal subspecies in its total distribution range (Huntington 1952; Yang and Selander 1968). The generalist nature and varied feeding strategies of Common Grackles, in conjunction with major anthropogenic changes in the North American landscape during the 18th and 19th Centuries, precipitated a rise in population numbers and their expansion into open agricultural regions as well as suburban parks and towns; population estimates rose to over 150 million by 1970 (Strassburg 2011). Since then, the species has undergone a gradual population reduction of > 50% which may be due to long standing lethal control methods aimed at protecting important agricultural products such as corn and sunflower from seasonal ravages of large flocks of grackles (Peer and Bollinger 2017). Although the range of the species is large, and the population is currently estimated at about 69, 000, 000 individuals, the International Union for Conservation of Nature Red List for Birds(2018, 2020) precautionarily lists the Common Grackle as a "Near Threatened" species (Bird Life International 2018, 2020a, 2020b). In this study, we focus on future (2070)distribution predictions of the Common Grackle to understand the role of climate change on its range dynamics.
Climatic modelling of the Common Grackle was completed using the R package Wallace (Kass et al. 2018) under the R v. 3.5.0 (R Core Team 2018) to model the species ecological niches for current and future (2070; ACCESS1-0, BCC-CSM1-1, CESM1-CAM5-1-FV2, CNRM-CM5, MIROC-ESM, and MPI-ESM-LR) bioclimatic conditions. The future (2070)bioclimatic conditions were based on the Representative Concentration Pathways 2.6 and 8.5 CO2 emissions pathway, long considered an 'optimistic scenario' and a 'worst case scenario', approaches that are the most appropriate for conducting assessments of climate change impacts by 2050 (Schwalm et al. 2020). In the modelling process, species occurrence data were compiled from our previous paper (Capainolo et al. 2020). Only breeding records of the Common Grackle between April and July of 2000-2019 were included and totaled more than 4 million records. Sampling bias and spatial autocorrelation were considered for occurrence records (Brown 2014). This allowed us to evaluate the effect of sampling bias on the ability of ecological niche modelling to project distributions into unsampled regions (Costa et al. 2010). Then, all records were filtered by eye for particularly unusual, unlikely, or spurious sightings, and artefacts were removed from the dataset. Occurrence records were spatially filtered within 25 km distances to remove duplicates and to get a more homogeneous dataset.
The WorldClim (version 1.4) bioclimatic variables were obtained at a resolution of 2.5 arcminutes (4.63 km at the equator). The background extent was set to "minimum convex polygon" with a 1-degree buffer distance and 10, 000 background points were sampled. We used block spatial partitioning. The model was built using the Maxent algorithm version 3.4.0 (Phillips et al. 2006; Elith and Leathwick 2009), using the feature classes: L (linear), LQ (linear quadratic), H (Hinge), LQH (Linear, Quadratic, Hinge), and LQHP (Linear, Quadratic, Hinge, Product) with the regularization multiplier 1 that is mostly considered the default setting in Maxent (Tomlinson et al. 2019).
The model was chosen based on the Akaike Information Criterion (AIC) score (Burnham and Anderson 2002). We also used AUC value, which is a threshold independent measure of predictive accuracy based only on the ranking of locations. The AUC value ranges from 0.5 to 1.0, where a value of < 0.5 describes no concordance with the data; in contrast, a 1.0 value indicates high model concordance, and values of > 0.6 imply good concordance. In general, the higher AUC value shows the higher performance of the model (Mousazade et al. 2019). With this method, we do not use high correlation bioclimatic variables, the threshold for the correlation coefficient was 0.85 based on the best model choice (for details, see Capainolo et al. 2020). The best models were then projected to the entire North American continent. We used the raster calculation option in ArcGIS version 10.2.2 to see the overlap area for the future predictions.
In total, 9804 occurrence records were used for ecological niche modelling after filtering. The best model performed was linear feature type with 1 regularization multiplier based on the Akaike information criterion corrected for small sample sizes (AICc=258808.4). The average training AUC for the replicate runs was 0.719, and the average test AUC for the replicate runs was 0.683. Since AUCs were larger than 0.6, model predictions were better than random predictions (see methods), that is, the model was significant for distributional predictions for the Common Grackle. The final models showed that BIO1 (Annual Mean Temperature), BIO2 (Mean Diurnal Range, mean of monthly max temp - min temp) BIO4 (Temperature Seasonality), BIO8 (Mean Temperature of Wettest Quarter), BIO 12 (Annual Precipitation) and BIO 15 (Precipitation Seasonality) are the most important variables for ecological niche modelling predictions for this species (Fig. 1).
Predictions under each of the six future global climate models showed that the Common Grackle will expand its range within the next 50 years (Figs. 2, 3). Both scenarios showed concordant patterns and there was no significant difference between the "optimistic" (RCP 2.6) and "worst-case" (RCP 8.5) predictions. The only difference was that the Common Grackle will expand its range slightly more under the "worst-case" scenario (Fig. 3).
A major challenge in understanding and predicting the impacts of climate change and global warming on biodiversity for the near future is to arrive at a generalizable and predictable understanding of these impacts. Studies modeling and predicting the effects of climate change on species distributions (Price 2000; Peterson et al. 2002) highlighted the specific and individual nature of the species' anticipated responses to climate change (Dunn and Møller 2019). In this study, we focused mostly on the future range dynamics of the Common Grackle and suggest that it will continue expanding northward and westward as well as possibly forming pioneering populations in the Caribbean and eastern Central America.
This study builds on the work of Capainolo et al. (2020) which showed that Common Grackles rapidly expanded their range north from a single refugium in southeastern North America as glaciers receded at the end of the last ice age. We looked at models of the near future (~50 years) under global warming scenarios for the likely distribution of the Common Grackle. There is clear evidence of a range expansion of the Common Grackle to the northern and western parts of the North American continent. Our future projection map (Fig. 3) shows expansion to the northwest into Alberta and British Columbia. The Rocky Mountains continue to act as an isolating barrier although there is evidence of a possible future patchy distribution of Common Grackle populations in the eastern foothills. Of interest is the novel occurrence of this species along the Pacific northwest coastline of the United States and Canada into coastal and southern Alaska. These regions may be colonized by birds using low altitude corridors to the west through British Columbia, some continuing along the coast north to Alaska and others moving south.
Many examples of vagile avian taxa traversing large distances, expanding their range, and colonizing new habitats are known (Crosby 1972; Bairlein et al. 2012; Gill et al. 2013; Oswald et al. 2019). The novel occurrence of 74 avian taxa in Alaska between 1976-1991 were documented by Gibson and Kessel (1992) including 20 species and 8 subspecies recorded for the first time in North America that include both non-passerine and passerine birds. Jamarillo and Burke (1999) state that, "Common Grackle is on the move in the western part of its distribution" and cite many records west of the Rocky Mountains including six from Alaska. Another Quiscalus grackle, the Great-tailed Grackle (Quiscalus mexicanus), has substantially expanded its range northward in North America since 1880 and benefits from human modified habitats and food sources (Wehtje 2003). These observations, records, and our model suggest an interesting possibility; the Common Grackle might eventually enter the Russian Far East by island hopping the Aleutians across the Bering Sea to become the first New World icterid to naturally colonize the Old World. It is almost certain that these western populations would be "Bronzed" grackles (Q. q. versicolor) as occasional vagrants recorded west of the Rockies are always this western subspecies (Jamarillo and Burke 1999; see www.ebird.org Common Grackle 2020).
Due to rapid expansion into isolated novel habitats, and sympatry with other icterid species such as the Red-winged Blackbird (Agelaius phoeniceus) (Lenington and Scola 1982) and Rusty Blackbird (Euphagus carolinus) (Matsuoka et al. 2010), it is possible that these pioneering populations of Common Grackle will rapidly adapt to new environments. Red-winged Blackbirds do not breed widely in Alaska, but Rusty Blackbirds do (Jaramillo and Burke 1999); possible competition for food in human-cleared habitats between Common Grackles and Rusty Blackbirds might negatively impact the latter (International Rusty Blackbird Technical Group 2009). Sympatry in the northwest breeding range of another icterid, the Brown-headed Cowbird (Molothrus ater), a significant brood parasite of numerous passerine bird species, is not likely to impact the breeding success of Common Grackles (Peer and Bollinger 1997b). The appearance of a new icterid species in the region might change the ecological dynamic via competition for food and nesting sites and perhaps, through natural selection, give rise to incipient forms of Common Grackle. Migrants from these regions might also establish the first winter populations west of the Rockies in suitable areas of Washington, Oregon, and California. Our projections also indicate possible coastal colonization in eastern Central America and islands in the Caribbean. These populations would probably be year-round non-migratory residents as are their likely progenitors, the nominate "Florida" Grackle (Q. q. quiscula).
The Common Grackle responded to climate change through Late Quaternary glacial-interglacial cycles and will respond to warming temperatures. Based on these results, this species will occupy a broader range than presently; we predict that the Common Grackle is a species that will benefit from slight changes in climate. This pattern has recently been shown for other avian taxa (e.g. Sharp-tailed Grouse Tympanuchus phasianellus; Perktaş and Elverici 2020, and Ruffed Grouse Bonassa umbellus; Perktaş 2021). For a broader range of bird species, see Peterson (2003).
Species distributions are strongly affected by climate, and climate change is largely affecting species and populations (Kreyling et al. 2015). Thermal niches have been widely used as proxies for estimating thermal sensitivity of species and have been frequently related to community composition, population trends and latitudinal/elevational shifts in distribution. In our analyses, temperature is one of the most important abiotic factors that affects the distribution of Common Grackles. Under the optimistic scenario (RCP 2.6), the Common Grackle will become more widespread in North America than it currently is. Our findings suggest that a moderate increase in temperature (RCP 2.6 is likely to keep global temperature increases below 2 ℃) is enough to cause range expansion into colder areas of the North American continent. Under the worst-case scenario, the Common Grackle will occupy Alaska, the only difference between the predictions of optimistic and worst-case scenarios. The temperature threshold and thermal sensitivity (Gaüzère et al. 2015) for the distribution of this species looks to be below 2 ℃, explaining why there is essentially no difference in distribution between the "best" and "worst" case scenarios in this study. A significant increase in distribution occurs with a 1 ℃ increase in temperature, but not with an additional 1 ℃ increase.
Our niche modelling predictions suggest that the Common Grackle, already a very widespread species, is likely to become even more so. However, these inferences are limited due to the confounding effects of biotic relations (Davis et al. 1998). This is an important limitation that could suggest that observed changes in niche characteristics were mostly dependent on the experimental conditions built into the models (Davis et al. 1998). To mitigate this, we used more than one global climate model in this study and considered the overlap of these predictions. Furthermore, the predicted increase in distribution in a warming world will by necessity depend on the future population trends of this species, and opportunity to expand distribution may well be limited by declining populations. This brings to the fore a relatively little discussed consequence of climate change. For some species, climate change will bring opportunity, but these opportunities can only be exploited if anthropogenic influences on populations are mitigated.
All possibilities that can be seen in the future distribution of Common Grackle are shown in the results; this study demonstrates that they will respond to global warming with significant range shift events. However, each of the past and future model outputs should be examined in more detail by including genetic data in the model outputs.
Common Grackles are an abundant New World blackbird that currently occupies a large home range east of the Rocky Mountains. A past study showed that the species responded to a naturally warming climate at the end of the last Ice Age and rapidly expanded its range to the north and west from refugia in the southeastern United States. They responded to massive human modifications to the environment in the eighteenth and nineteenth centuries; clearing of the great eastern forests and the planting of cash crops caused an increase in population number and range shifts into newly open and semi-open habitats. A population decline began in the 1970's and may be due to lethal control measures aimed at protecting agricultural interests; the current population is estimated at around 69, 000, 000. Common Grackles are temperature sensitive and respond to warming temperatures by expanding their range into regions formerly too cold for them to thrive in. The modelling in this study suggests further expansion into regions of northwestern North America (and other areas not currently occupied by Common Grackles) under warming temperature scenarios over the next 50 years.
We thank editor Federico Morelli and two anonymous reviewers for valuable insights and recommendations which greatly improved the manuscript.
PC, UP & MDE, fellowes contributed equally to all aspects of this study. All authors read and approved the final manuscript.
Not applicable.
The datasets used in the present study are available from the corresponding author on reasonable request.
Not applicable.
Not applicable.
The authors declare that they have no competing interests.
Akcakaya HR, Sjögren-Gulve P. Population viability analyses in conservation planning: an overview. Ecol Bull. 2000;48: 9–21.
|
Chen JP. Allee effects based on night-roosting flock, individual survival, population growth for the reintroduced popuation of the Crested Ibis (Nipponia nippon) in Ningshan, Shaanxi Province, China. Master's Thesis. Shaanxi: Shaanxi Normal University; 2018 (in Chinese).
|
Dong R, Ye XP, Zhong L, Li X, Li M, Wang HQ, et al. Effects of breeding success, age and sex on breeding dispersal of a reintroduced population of the Crested Ibis (Nipponia nippon) in Ningshan County, China. Avian Res. 2018;9: 314–20.
|
IUCN/SSC. Guidelines for reintroductions. Gland: IUCN Species Survival Commission; 1998.
|
IUCN/SSC. Guidelines for reintroductions and other conservation translocations. Version 1.0. Gland: IUCN Species Survival Commission; 2013.
|
Lande R, Barrowclough GF. Effective population size, genetic variation, and their use in population management. In: Soulé ME, editor. Viable populations for conservation. Cambridge: Cambridge University Press; 1987. p. 87–124.
|
Li HY. A population viability analysis for Nipponia nippon. Master's Thesis. Beijing: Beijing Forestry University; 2013 (in Chinese).
|
Macdonald DW, Johnson DDP. Dispersal in theory and practice: consequences for conservation biology. In: Clobert J, Danchin E, Dhondt AA, Nichols JD, editors. Dispersal. Oxford: Oxford University Press; 2001. p. 358–72.
|
Miller PS, Lacy RC. VORTEX: a stochastic simulation of the extinction process. Version 9.5.0 user's manual. Apple Valley: Conservation Breeding Specialist Group (SSC/IUCN); 2005.
|
Morris WF, Doak DF. Quantitative conservation biology: theory and practice of population viability analysis. Sunderland: Sinauer Associates; 2002.
|
Nagata H, Yamagishi S. Which factors affect post-release settlement of Crested Ibis Nipponia nippon on Sado Island, Japan? Ornithol Sci. 2016;15: 181–9.
|
Reed JM, Murphy DD, Brussard PF. Efficacy of population viability analysis. Wildl Soc Bull. 1998;26: 244–51.
|
Wang M. Outcome assessment of the reintrodution of the Crested Ibis (Nipponia nippon) implemented in Ningshan County, Shaanxi, China. Master's Thesis. Shaanxi: Shaanxi Normal University; 2015 (in Chinese).
|
Wei D. Habitat suitability evaluation of reintroduced Crested Ibis from Shaanxi province. Master's Thesis. Shaanxi: Shaanxi Normal University; 2020 (in Chinese).
|
Zhai TQ, Wang ZY, Zhang HJ. The study on the reproduction of two ages Crested Ibis (Nipponia nippon). Acta Ecol Sin. 1994;14: 99–101 (in Chinese).
|
1. | Jeremy Summers, Dieter Lukas, Corina J Logan, et al. The role of climate change and niche shifts in divergent range dynamics of a sister-species pair. Peer Community Journal, 2023, 3 DOI:10.24072/pcjournal.248 |
2. | Marta Kolanowska. Future distribution of the epiphytic leafless orchid (Dendrophylax lindenii), its pollinators and phorophytes evaluated using niche modelling and three different climate change projections. Scientific Reports, 2023, 13(1) DOI:10.1038/s41598-023-42573-5 |
3. | Peter Capainolo, Utku Perktaş, Can ElverıCı, et al. Subspecies limits based on morphometry and mitochondrial DNA genomics in a polytypic species, the common grackle, Quiscalus quiscula. Biological Journal of the Linnean Society, 2023, 139(1): 39. DOI:10.1093/biolinnean/blad009 |