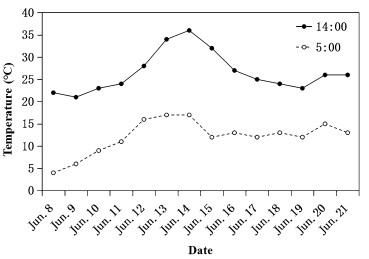
Citation: | Justin J. Reel, Todd J. Underwood. 2019: Egg rejection behavior does not explain the lack of cowbird parasitism on an eastern North American population of Red-winged Blackbirds. Avian Research, 10(1): 47. DOI: 10.1186/s40657-019-0186-1 |
Red-winged Blackbirds (Agelaius phoeniceus),hereafter red-wings,are much less frequently parasitized by Brown-headed Cowbirds (Molothrus ater) in eastern North America than in central North America and had not been recorded as hosts in our study area in southeastern Pennsylvania. Although hosts of Old World cuckoos (Cuculidae) often show geographic variation in egg rejection behavior,cowbird hosts typically exhibit uniform responses of all acceptance or all rejection of cowbird eggs. Thus,geographic variation in cowbird parasitism frequencies might reflect a different behavioral response to parasitism by hosts where only some populations reject parasitism. In this study,we tested whether egg rejection behavior may explain the lack of parasitism observed in our eastern red-wing population,which may provide insight into low parasitism levels across eastern North America.
We parasitized red-wing nests with model cowbird eggs to determine their response to parasitism. Nests were tested across three nest stages and compared to control nests with no manipulations. Because rejection differed significantly by stage,we compared responses separately for each nest stage. We also monitored other songbird nests to identify parasitism frequencies on all potential hosts.
Red-wings showed significantly more rejections during the building stage,but not for the laying and incubation stages. Rejections during nest building involved mostly egg burials,which likely represent a continuation of the nest building process rather than true rejection of the cowbird egg. Excluding these responses,red-wings rejected 15% of cowbird eggs,which is similar to rejection levels from other studies and populations. The overall parasitism frequency on 11 species surveyed in our study area was only 7.4%.
Egg rejection behavior does not explain the lack of parasitism on red-wings in our eastern population. Alternatively,we suggest that cowbird preference for other hosts and the low abundance of cowbirds in the east might explain the lack of parasitism. Future research should also explore cowbird and host density and the makeup of the host community to explain the low levels of parasitism on red-wings across eastern North America because egg rejection alone is unlikely to explain this broad geographic trend.
In temperate zone, passerine birds time their breeding to track the availability of their food (Lack 1968), because birds have to raise their young at times with highest food abundance to achieve the highest reproductive output (Nager and van Noordwijk 1995; Dias and Blondel 1996). Many studies have found higher food availability results in increased growth rates (Keller and van Noordwijk 1993), and larger fledgling mass improves offspring survival (Gebhardt-Henrich and van Noordwijk 1991; Verboven and Visser 1998). The underlying mechanism of this relationship between food availability and offspring fitness remains largely unexplored. Some studies have explained this relationship from the point of malnutrition. It has been found in some passerine bird species such as Great Tit (Parus major) that the optimal food (e.g. Lepidoptera) has higher quality nutritional profile than the alternative food (e.g. Diptera and Coleoptera) (Arnold et al. 2010). Some studies found plasma metabolite level can predict changes in the individual body condition, growth rates and survival rate of nestlings (Nadolski et al. 2006; Amat et al. 2007; Albano et al. 2011). However, there is little work to reveal the physiological responses of the nestlings hatched asynchronized with the food abundance peak, which is important for understanding the causal link between the timing of breeding and the fitness of offspring.
Food shortage is a major environmental factor inducing stress of wild animals (Merino et al. 2002; Herring et al. 2011). Understanding the stress status of the nestlings hatched in different food conditions is important to reveal the biological mechanism linking the food availability and fitness of offspring. Cells are the fundamental units of organisms, and the survival of the whole organism depends on the ability of cells to maintain homeostasis. The cellular stress response, an important molecular mechanism involved in maintaining cellular homeostasis, can offset the effects of stress and thereby allow other processes to restore cellular homeostasis (Kultz 2005; Somero 2010). Heat shock proteins (HSPs) are molecular chaperones that help maintain regular cellular functions by playing a crucial role in protein folding, unfolding, aggregation, degradation and transport (Beissinger and Buchner 1998; Sørensen et al. 2003; Arya et al. 2007). HSPs increase in response to acute and chronic stress, injury, and disease (Santoro 2000; Al-Aqil et al. 2013; Zulkifli et al. 2014). In particular, HSP60, 70 and 90 have been shown to be indicators of stress in several avian species, including Barn Swallows (Hirundo rustica), Broiler Chickens, and House Finches (Haemorhous mexicanus) (Merino et al. 2002; Beloor et al. 2010; Hill et al. 2013; Wein et al. 2016). Therefore, the levels of HSPs can be used as markers to reflect physiological status of the nestlings hatched under different food condition.
Asian Short-toed Lark (Calandrella cheleensis) is the most common passerine species living on the grasslands of northeastern Inner Mongolia of China (Tian et al. 2015; Zhang et al. 2017). The breeding of the species begins in mid-April and ends in mid-June, and the grasshopper nymph (Orthoptera) is the main component (about 80%) of the nestlings' diet (Tian et al. 2015). Because there is only one significant nymph abundance peak once a year in the study area (Chen and Gong 2005; Guo et al. 2009), and the hatching time of nestlings has to be synchronized with the time of nymph abundance peak. According to our previous study, the species displays significantly within year individual variation in the timing of breeding behavior (Zhang et al. 2017; Zhao et al. 2017). Although there was an obvious hatching peak in one breeding season, there were some nestlings hatched outside this peak (Zhang et al. 2017). In addition, the synchronization between the nestling hatching peak and nymph abundance peak varied with the spring temperature. In 2014 and 2016, the peak of nestling hatching was asynchronized with the nymph abundance peak for the extremely high and low spring temperature, and nestling's food shortage induced by such mismatch could finally reduce the survival rate of nestlings (Zhang et al. 2017). Therefore, this species is an appropriate species to study the effect of food availability on the physiological status of nestlings.
In this paper, we hypothesize that the nestlings of Asian Short-toed Lark hatched under poor optimal food condition would activate cell stress response. To test this hypothesis, we compared the gene expression of HSP70 and HSP90 in the blood cells of Asian Short-toed Lark nestlings hatched under different nymph abundance conditions in 2017.
Our study area was in the Hulun Lake National Nature Reserve (47°45′50″‒49°20′20″N, 116°50′10″‒118°10′10″E), which is in the northeastern part of the Inner Mongolian Autonomous Region, China. This is a semiarid, steppe region where the mean annual temperature, precipitation and potential evaporation are - 0.6 ℃, 283 mm and 1754 mm, respectively. The dominant plant species are Stipa krylovii, Leymus chinesis and Cleistogenes squarrosa. Winter is longer than summer and the approximate average maximum daytime temperatures in January and July are - 20.02 ℃ and 22.72 ℃, respectively.
Asian Short-toed Larks nest on the ground. From 20 April to 27 June in 2017, we surveyed nests of larks and grasshopper nymph in the study area every day. From 8 June to 21 June, we took blood samples of 64 four-day-old nestlings from different nests and recorded their hatching date. Considering the daily temperature variation (Fig. 1), each sampled nestling was blooded on 5:00 and 14:00 respectively. The relative abundance of grasshopper nymphs in the study area was quantified by catching them in an insect net on 10 parallel, 2 m × 100 m sampling transects, spaced 10 m apart, every 2 days. Captured nymphs were dried and weighed to determine their biomass. The mean daily nymph biomass was the average daily biomass obtained from all 10 transects. Annual variations in the timing of hatching and daily nymph biomass are shown in Fig. 2.
About 100 μL of whole blood was collected from 64 four-day-old nestlings to measure HSP70 and HSP90 gene expression levels. A brachial wing vein of each nestling was punctured with a disinfected 23 G needle within 1-3 min of capture and blood that exuded from the puncture site was collected into heparinized microcapillary tubes. The skin around the puncture site was disinfected with medical alcohol before, and after, puncturing. Pressure was applied to the puncture site for 1 min with an alcohol-soaked cotton wool swab to staunch bleeding. Blood sampling procedures complied with ARRIVE guidelines and were approved by the Animal Research Ethics Committee of the Hainan Provincial Education Centre for Ecology and Environment, Hainan Normal University (permit no. HNECEE-2013-002). The study is carried out with the permissions of Hulun Lake National Nature Reserve.
Total RNA from the blood samples was isolated from 1 mL TRIzol reagent in 200 μL chloroform, centrifuged at 12, 000 r/min for 15 min at 4 ℃ after which about 500 μL of the supernatant was transferred to a clean tube, adding 500 μL isopropanol to the tube and centrifuging at 12, 000 r/min for 15 min at 4 ℃. The resultant RNA pellet was washed with 1 mL 75% ethanol (dissolved in DEPC water) two times. The RNA pellet was briefly air dried for 5 min and dissolved in 30 μL DEPC water. Total RNA quality and quantity were evaluated using agarose gel electrophoresis (AGE) and a NanoDrop 2000 spectrophotometer, and 2 μg total RNA sample was reversely transcribed to cDNA using M-MLV Reverse Transcriptase with oligo dT primers. The cDNA was used as a template in RT-qPCR. Primers were designed based on HSP70 and HSP90 mRNA sequences in NCBI using Primer3 software (Table 1). The procedures for RT-qPCR were as follows: pre-degeneration at 95 ℃ for 1 min, degeneration at 95 ℃ for 15 s, annealing at 59 ℃ for 15 s and extension at 72 ℃ for 40 s, totally 40 cycles. After the reaction had completed, a melting curve analysis from 55 to 95 ℃ was applied to ensure consistency and specificity of the amplified product. The expression of β-actin was confirmed to be stable under all treatments and this gene was consequently used as the reference gene to normalize mRNA levels among samples. RT-qPCR was performed twice, in triplicate. The values of the average cycle threshold (Ct) were determined and Ct scores for gene transcripts in each sample were normalized using the ΔCt scores for β-actin and expressed as the fold change in gene expression using the equation 2-ΔΔCT.
Gene | Forward primer | Size (bp) | Accession no. |
HSP70 | F:5-TTTCCTTGTGCCCTGCTGTA-3 R: 5-ACCTAACTTGTCCCAAAACTGC-3 |
163 | AY466445 |
HSP90 | F:5-CGATTGGTTGGTCCTGAGTT-3 R:5-AAGGTTCCCGATGCTTCTG-3 |
186 | HQ162267 |
β-actin | F:5-CACAATGTACCCTGGCATTG-3 R:5-ACATCTGCTGGAAGGTGGAC-3 |
158 | L08165 |
We used "nymph biomass proportion (NBP)", the percentage of the daily nymph biomass relative to the total biomass measured over all survey days, as a measure of grasshopper nymph abundance in the statistical analysis. The effects of nymph biomass, sample time and their interaction on the blood HSP70 and HSP90 gene expression levels in nestlings are analyzed with a linear mixed model (LMM) in which the individual nestling is a random factor. Regression analysis is used to analyze the relationship between nymph biomass and the gene expression level of HSP70 and HSP90 if the effect of nymph biomass is significant in the LMMs. We used α = 0.05 as the significant level for all statistical tests. All analyses were performed using SPSS version 24 (SPSS Inc., Chicago, USA).
The results of the LMMs for the effects of nymph biomass and sample time on gene expression level of HSP70 and HSP90 indicate that nymph biomass, sample time and interaction of the two factors significantly influenced the blood HSP70 and HSP90 gene expression level of Asian Short-toed Lark nestlings (Table 2). According to this result, the relationships between nymph biomass and HSP70 and HSP90 gene expression level at 5:00 and 14:00 were analyzed separately. Simple linear regression analysis showed that the nymph biomass negatively correlated with the gene expression level of HSP70 (5:00, n = 64, R2 = 0.811, Fig. 3a; 14:00, n = 64, R2 = 0.636, Fig. 3c) and HSP90 (5:00, n = 64, R2 = 0.666, Fig. 3b; 14:00, n = 64, R2 = 0.743, Fig. 3d). The gene expression levels of HSP70 and HSP90 increase with the decrease of nymph biomass (Fig. 3). In addition, both HSP70 and HSP90 gene expression levels at 14:00 are significantly higher than those at 5:00 (Fig. 3).
HSPs | Explanatory variable | F | p |
HSP70 | Nymph biomass | 88.36 | < 0.001 |
Sample time | 213.65 | < 0.001 | |
Nymph biomass × sample time | 4.77 | < 0.001 | |
HSP90 | Nymph biomass | 99.49 | < 0.001 |
Sample time | 265.12 | < 0.001 | |
Nymph biomass × sample time | 8.97 | < 0.001 |
The LMM results indicate that the nymph abundance is a factor which significantly influences the gene expressions of HSP70 and HSP90 of Asian Short-toed Lark nestlings. Negative correlation between nymph biomass and gene expressions of HSP70 and HSP90 suggests that poor food condition is an important environment factor inducing cell stress of Asian Short-toed Lark nestlings. Similar results about the relationship between food availability and stress protein level of nestlings have been found in other species such as White Ibis which showed that the HSP60 levels increased predictably in response to food limitation (Herring and Gawlik 2013). Our results and the available study jointly show that food shortage during post embryo development can induce the stress in nestlings. Several studies have compared the response of HSP60, HSP70 and HSP90 to the ecological factors. While some of the studies found HSP60 is more sensitive than other HSPs (Martínez-Padilla et al. 2004; Moreno et al. 2005), other studies found HSP70 was more sensitive to environmental perturbations than HSP60 or HSP90 (Ulmasov et al. 1992; Fader et al. 1994; McMillan et al. 2011). Our study showed that HSP70 and HSP90 of nestlings respond to the optimal food condition similarly. The different responses of the HSPs might be species specific, therefore we suggest that multiple HSPs should be used as cell stress markers in the evaluation of cell stress status.
In addition to the food availability, the LMM results of our study also indicate the sample time is also an important factor inducing the variation of HSPs level. HSP70 and HSP90 gene expressions in the blood sampled at 14:00 were significantly higher than those sampled at 5:00, which could be explained by the daily temperature variation in the habitat. Our study area was in the northeastern part of the Inner Mongolian Autonomous Region, where the daily temperature variation between minimum and maximum is 10‒15 ℃ (Chen and Gong 2005). The temperature at 14:00 was higher than that at 5:00 (Fig. 1). Temperature variation has been found to be an important environment factor inducing the HSPs expression (Arya et al. 2007). Therefore, the expression level of HSPs at 14:00 increasing in our study indicates the nestlings could cope with the temperature variation to maintain homeostasis by cell stress response. The response of blood HSPs to the marked temperature variation in the study area has been found in our previous study of adult Asian Short-toed Larks (Qin et al. 2017). These results indicate that cell stress response is an important physiological mechanism for birds to cope with highly stochastic ecosystems not only in adult but also in nestlings. Our LMM model suggested that poor food condition enhanced the level of cell stress of nestlings at both low and high temperature.
Although the HSPs can maintain the homeostasis of the cells, overexpression of these stress proteins is known to have deleterious consequences (Feder and Hofmann 1999). Their synthesis represents a significant energetic cost (Hamdoun et al. 2003), which can lead to reduced fecundity (Parsons 1996). In addition, their response usually results in a concomitant reduction in the synthesis of antibodies, thus synthesizing more HSPs to mitigate stress has been found in passerine birds to be traded-off against mounting humoral and cell-mediated immune responses (Morales et al. 2006). The result of our previous study on the Asian Short-toed Lark showed that the survival rate of the nestlings in 2014 and 2016 when the peak of nestling hatching mismatched with the peak of nymph abundance peak was significantly lower than that in 2015 when the two peaks thoroughly match (Zhang et al. 2017), which suggests that overexpression of HSPs could be related with lower nestling survival. As a bird species living in the harsh weather condition, the nestlings of Asian Short-toed Lark have to cope with the marked weather variation by cell stress response, and poor food condition could impose additional stress to make HSPs overexpression, which consequently could result in negative effect on the nestling survival. Therefore, over cell stress response may be one of physiological factor mediating the effect of optimal food availability and the nestling's survival.
Shifts in phenology induced by climate change are altering ecological relationships and processes around the world (Visser and Both 2005; Cleland et al. 2007; Forrest and Miller-Rushing 2010) and there is a growing body of literature on resultant trophic mismatches between nestlings and their food resources (Visser et al. 1998; Visser and Both 2005; Thackeray et al. 2010). Although such trophic mismatches have been found to result in nestlings having lower body mass, smaller body size and reduced survival rates (Verboven and Visser 1998; Visser et al. 1998; Durant et al. 2007; Ovaskainen et al. 2013; Dunn and Møller 2014), there is little information to reveal the physiological mechanism how the optimal food condition influences the fitness of the nestlings. Our research indicates that cell stress biomarkers have the potential to respond directly and predictably to optimal food conditions. Under the phenological mismatch scenario, the birds living in the fluctuating environment would encounter more stress challenges, which implicates that these birds could be more vulnerable to the phenological mismatch. Therefore, we suggest the vulnerability of the species to the phenological mismatch induced by climate change should be estimated combining the physiological and ecological data.
The results of this study indicate that poor food condition is an important environment factor inducing cellular stress of Asian Short-toed Lark nestlings. The interactive effect of the nymph abundance and sample time on the HSPs response may be related with the daily temperature variation of the grassland. Over cell stress response may be one of physiological factor mediating the effect of food availability and the nestling's fitness.
We are grateful to Manquan Gui, Muren Wu, Songtao Liu in Hulun Lake National Nature Reserve for their help on the field study.
SZ and WL conceived the study and designed the experiments. L. Zhaang, L. Zhao and XZ conducted the experiments. LZ wrote the first draft of the article. SZ supervised the research and revised the draft. All authors read and approved the final manuscript.
The data used in the present study are available from the corresponding author on reasonable request.
Our experimental procedures complied with the current laws on animal welfare and research in China and had the approval of the Animal Research Ethics Committee of Hainan Normal University. In addition, all procedures followed standard protocols, such as the ARRIVE guidelines for reporting animal research.
The authors declare that they have no competing interests.
Not applicable.
Berger AJ. The cowbird and certain host species in Michigan. Wilson Bull. 1951;63:26-34.
|
Blankespoor GW, Oolman J, Uthe C. Eggshell strength and cowbird parasitism of Red-winged Blackbirds. Auk. 1982;99:363-5.
|
Campbell RW, Dawe NK, McTaggart-Cowan I, Cooper JM, Kaiser GW, Stewart AC, et al. The birds of British Columbia, Vol. 4. Vancouver: UBC Press; 2001.
|
Cannings RA, Cannings RJ, Cannings SG. Birds of the Okanagan Valley, British Columbia. Victoria: Royal British Columbia Museum; 1987.
|
Carello CA, Snyder G. The effects of host numbers on cowbird parasitism of Red-winged Blackbirds. In: Smith JNM, Cook TL, Rothstein SI, Robinson SK, Sealy SG, editors. Ecology and management of cowbirds and their hosts. Austin: University of Texas Press; 2000. p. 110-4.
|
Ely CA. Comparative nesting success of certain south-central Oklahoma birds [M.S. thesis]. Norman: University of Oklahoma; 1957.
|
Feeney WE. Evidence of adaptations and counter-adaptations before the parasite lays its egg: the frontline of the arms race. In: Soler M, editor. Avian brood parasitism: behavior, ecology, evolution and coevolution. Cham: Springer; 2017. p. 307-24.
|
Fleischer RC. Brood parasitism by Brown-headed Cowbirds in a simple host community in eastern Kansas. Kansas Ornithol Soc Bull. 1986;37:21-9.
|
Friedmann H, Kiff LF, Rothstein SI. A further contribution of knowledge of the host relations of the parasitic cowbirds. Smithson Contr Zool. 1977;235:1-75.
|
Goertz JW. Additional records of Brown-headed Cowbird nest parasitism in Louisiana. Auk. 1977;94:386-9.
|
Hanka LR. Choice of host nest by the Brown-headed Cowbird in Colorado and Wyoming. Condor. 1979;8:436-7.
|
Hill RA. Host-parasite relationships of the Brown-headed Cowbird in a prairie habitat of west-central Kansas. Wilson Bull. 1976;88:555-65.
|
Koford RR, Bowen BS, Lokemoen JT, Kruse AD. Cowbird parasitism in grassland and cropland in the northern Great Plains. In: Smith JNM, Cook TL, Rothstein SI, Robinson SK, Sealy SG, editors. Ecology and management of cowbirds and their hosts. Austin: University of Texas Press; 2000. p. 229-35.
|
Linz GM, Bolin SB. Incidence of Brown-headed Cowbird parasitism on Red-winged Blackbirds. Wilson Bull. 1982;94:93-5.
|
Lowther PE. Old cowbird breeding records from the Great Plains. Bird Band. 1977;48:358-69.
|
Lowther PE. Chickadee, thrasher, and other cowbird hosts from northwest Iowa. J Field Ornithol. 1983;54:414-7.
|
McWilliams GM, Brauning DW. The birds of Pennsylvania. Ithaca: Comstock; 2000.
|
Middleton ALA. Effect of Cowbird parasitism on American Goldfinch nesting. Auk. 1977;2000(94):304-7.
|
Moksnes A, Røskaft E, Braa AT. Rejection behavior by Common Cuckoo hosts towards artificial brood parasite eggs. Auk. 1991;108:348-54.
|
Orians GH, Røskaft E, Beletsky LD. Do Brown-headed Cowbirds lay their eggs at random in the nests of Red-winged Blackbirds? Wilson Bull. 1989;101:599-605.
|
Ortega CP. The ecology of blackbird/cowbird interactions in Boulder County, Colorado [dissertation]. Bolder: University of Colorado; 1991.
|
Ortega CP. Cowbirds and other brood parasites. Tucson: University of Arizona Press; 1998.
|
Peck GK, James RD. Breeding birds of Ontario: nidiology and distribution—volume 2: passerines. Toronto: Royal Ontario Museum; 1987.
|
Peterjohn BG, Sauer JR, Schwartz S. Temporal and geographic patterns in population trends in brown-headed cowbirds. In: Smith JNM, Cook TL, Rothstein SI, Robinson SK, Sealy SG, editors. Ecology and management of cowbirds and their hosts. Austin: University of Texas Press; 2000. p. 21-34.
|
Robbins CS, Blom EAT. Atlas of the breeding birds of Maryland and the District of Columbia. Pittsburgh: University of Pittsburgh Press; 1996.
|
Robinson SK, Hoover JP, Herkert JR. Biogeographic, landscape, and local factors affecting cowbird abundance and host parasitism levels. In: Smith JNM, Cook TL, Rothstein SI, Robinson SK, Sealy SG, editors. Ecology and management of cowbirds and their hosts. Austin: University of Texas Press; 2000. p. 280-97.
|
Rothstein SI, Peer BD. Conservation solutions for threatened and endangered cowbird (Molothrus spp.) hosts: separating fact from fiction. Ornithol Monogr. 2005;57:98-114.
|
Ruiz-Raya F, Soler M. Phenotypic plasticity in egg rejection: evidence and evolutionary consequences. In: Soler M, editor. Avian brood parasitism: behavior, ecology, evolution and coevolution. Cham: Springer; 2017. p. 449-71.
|
Sealy SG, Banks AJ, Chace JF. Two subspecies of Warbling Vireo differ in their response to Cowbird eggs. West Birds. 2000;31:190-4.
|
Smith HM. Irregularities in the egg laying behavior of the eastern cowbird. J Colorado-Wyoming Acad Sci. 1949;4:60.
|
Southern WE, Southern LK. A summary of the incidence of cowbird parasitism in northern Michigan from 1911-1948. Jack-Pine Warbler. 1980;58:77-84.
|
Terrill LM. Cowbird hosts in southern Quebec. Can Field-Nat. 1961;75:2-11.
|
Thompson FR III, Robinson SK, Donovan TM, Faaborg J, Whitehead DR, Larsen DR. Biogeographic, landscape, and local factors affecting cowbird abundance and host parasitism levels. In: Smith JNM, Cook TL, Rothstein SI, Robinson SK, Sealy SG, editors. Ecology and management of cowbirds and their hosts. Austin: University of Texas Press; 2000. p. 271-9.
|
Uhrich WD. A century of bird life in Berks County, Pennsylvania. Reading: Reading Public Museum; 1997.
|
Underwood TJ, Sealy SG. Grasp-ejection in two small ejecters of cowbird eggs: a test of bill-size constraints and the evolutionary equilibrium hypothesis. Anim Behav. 2006a;71: 409-16.
|
Underwood TJ, Sealy SG. Parameters of Brown-headed Cowbird Molothrus ater egg recognition and ejection in Warbling Vireos Vireo gilvus. J Avian Biol. 2006b;37: 457-66.
|
Walkinshaw LH. Brown-headed Cowbird Molothrus ater. In: Brewer R, McPeek GA, Adams Jr RJ, editors. The atlas of breeding birds in Michigan. East Lansing: Michigan State University Press; 1991. p. 508-9.
|
Ward D, Smith JNM. Interhabitat differences in parasitism frequencies by Brown-headed Cowbirds in the Okanagan Valley, British Columbia. In: Smith JNM, Cook TL, Rothstein SI, Robinson SK, Sealy SG, editors. Ecology and management of cowbirds and their hosts. Austin: University of Texas Press; 2000. p. 210-9.
|
Weatherhead PJ. Sex ratios, host-specific reproductive success, and impact of Brown-headed Cowbirds. Auk. 1989;106:358-66.
|
Wiens JA. Aspects of cowbird parasitism in southern Oklahoma. Wilson Bull. 1963;75:130-9.
|
Yasukawa K, Werner W. Nest abandonment as a potential anti-parasite adaptation in the red-winged blackbird. Passeng Pigeon. 2007;69:481-90.
|